The following is an excerpt from Extended Heredity: A New Understanding of Inheritance and Evolution by Russell Bonduriansky and Troy Day.
The idea that genes encode all the heritable features of living things has been a fundamental tenet of genetics and evolutionary biology for many years, but this assumption has always coexisted uncomfortably with the messy findings of empirical research. The complications have multiplied exponentially in recent years under the weight of new discoveries.
Classical genetics draws a fundamental distinction between the “genotype” (that is, the set of genes that an individual carries and can pass on to its descendants) and the “phenotype” (that is, the transient body that bears the stamp of the environments and experiences that it has encountered but whose features cannot be transmitted to offspring). Only those traits that are genetically determined are assumed to be heritable—that is, capable of being transmitted to offspring—because inheritance occurs exclusively through the transmission of genes. Yet, in violation of the genotype/phenotype dichotomy, lines of genetically identical animals and plants have been shown to harbor heritable variation and respond to natural selection.
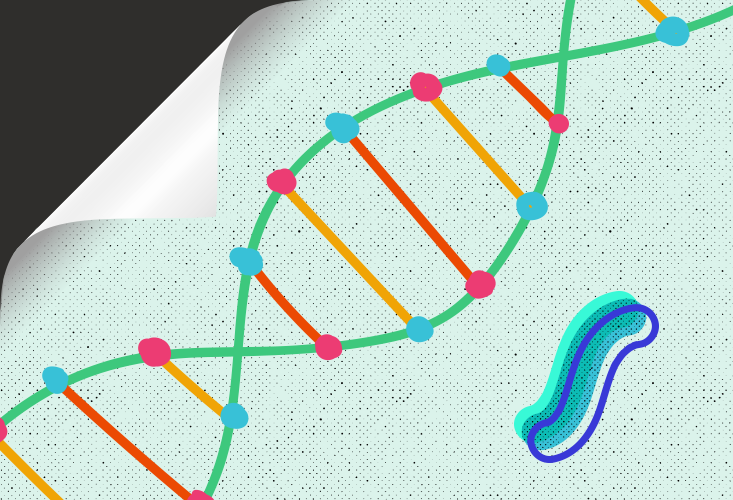
Conversely, genes currently fail to account for resemblance among relatives in some complex traits and diseases—a problem dubbed the “missing heritability.”1 But, while an individual’s own genotype doesn’t seem to account for some of its features, parental genes have been found to affect traits in offspring that don’t inherit those genes. Moreover, studies on plants, insects, rodents, and other organisms show that an individual’s environment and experiences during its lifetime—diet, temperature, parasites, social interactions—can influence the features of its descendants, and research on our own species suggests that we are no different in this respect. Some of these findings clearly fit the definition of “inheritance of acquired traits”—a phenomenon that, according to a famous analogy from before the Google era, is as implausible as a telegram sent from Beijing in Chinese arriving in London already translated into English. But today such phenomena are regularly reported in scientific journals. And just as the Internet and instant translation have revolutionized communication, discoveries in molecular biology are upending notions about what can and cannot be transmitted across generations.
Biologists are now faced with the monumental challenge of making sense of a rapidly growing menagerie of discoveries that violate deeply ingrained ideas. One can get a sense of the growing dissonance between theory and evidence by perusing a recent review of such studies and then reading the introductory chapter from any undergraduate biology textbook. Something is clearly missing from the conventional concept of heredity, which asserts that inheritance is mediated exclusively by genes and denies the possibility that some effects of environment and experience can be transmitted to descendants.
If some nongenetic variation is heritable, then it follows that such variation can respond to natural selection and produce phenotypic change across generations in the absence of genetic change. Such changes do not fit the standard genetic definition of evolution, which is restricted to change in allele frequencies across generations. This definition, devised by evolutionary geneticist Theodosius Dobzhansky, rejected the assumption that genes are the only source of heritable variation, and therefore the only raw material on which natural selection could act to produce phenotypic change across generations. However, it’s useful to recall that Charles Darwin was blissfully unaware of the distinction between genetic and nongenetic variation. Darwin’s profound insight was that natural selection, applied to heritable variation within a population, can produce change across generations in the average features of organisms because those heritable traits that are consistently associated with a larger number of surviving offspring will be represented in a greater proportion of individuals in each generation.2 The incorporation of nongenetic mechanisms into heredity does not require any change in this basic Darwinian equation.
One category of nongenetic effects—maternal effects—are so obvious that their existence has been recognized for several decades. By definition, maternal effects occur when maternal phenotype affects offspring phenotype, and this effect cannot be explained by the transmission of maternal alleles.3,4 Such effects can occur via the myriad routes of influence that mothers have on their offspring, including transgenerational epigenetic inheritance, variation in the structure of the egg cell, the intrauterine environment, the mother’s choice of the location where the eggs or offspring are brought into the world, and modification of the environment that the offspring will experience, as well as postpartum physiological and behavioral interactions. Some maternal effects are passive consequences of maternal features for offspring development (including deleterious effects of maternal poisoning, illness, or senescence), while other maternal effects represent reproductive investment strategies that have evolved to enhance reproductive success.4,5 Such effects can therefore either enhance or reduce the fitness of mothers and their offspring.
Until quite recently,6 maternal effects were regarded as little more than a nuisance—a source of environmental “error” in genetic studies. But geneticists at least felt assured that, in most species (including key laboratory “model organisms” such as flies and mice), fathers could transmit nothing more than genetic alleles to their offspring.7 Yet, recent research has revealed numerous examples of paternal effects in mice, Drosophila, and many other species as well.8 In fact, in sexually reproducing species, paternal effects could turn out to be as widespread as maternal effects.
Discoveries in molecular biology are upending notions about what can and cannot be transmitted across generations.
Offspring can be influenced by the environment and experience, age, and genotype of both parents. An environmental factor (such as a toxin or a nutrient) could induce changes in the parental body that affect offspring development. As we will see, the deterioration of the body with age can also affect reproductive traits and heritable nongenetic factors, and thereby affect offspring development.
Cases where a gene expressed in a parent influences the phenotype of its offspring are known as “indirect genetic effects.”9 Perhaps counter-intuitively, such effects fit within the scope of nongenetic inheritance because they are mediated by the transmission of nongenetic factors. For example, a particular gene expressed in a parent might affect its behavior toward its offspring, or alter the epigenetic profile of other genes in the germ line, and thereby affect the development of offspring even if they do not inherit that gene.
A striking example of an indirect genetic effect was provided by a study on mice. Vicki Nelson and colleagues crossed different inbred mouse strains to produce males that were genetically similar in every way except for their Y chromosome. They then asked a very odd question: Does a male’s Y chromosome affect the phenotype of his daughters? Anyone who stayed awake through their high-school biology classes knows that daughters don’t inherit their father’s Y chromosome, so, by the logic of classical genetics, genes on the paternal Y chromosome cannot affect daughters. But Nelson and colleagues found that the identity of the paternal Y chromosome did affect a variety of physiological and behavioral traits in daughters. In fact, the effects of the paternal Y chromosome on daughters were comparable in magnitude to the effects of the paternal autosomes or X chromosome, which daughters do inherit. Although the mechanism involved remains unknown, genes on the Y chromosome must have somehow altered the sperm cytoplasm, sperm epigenome, or the composition of the seminal fluid, allowing genes on the Y chromosome to influence the development of offspring that did not inherit those genes.10
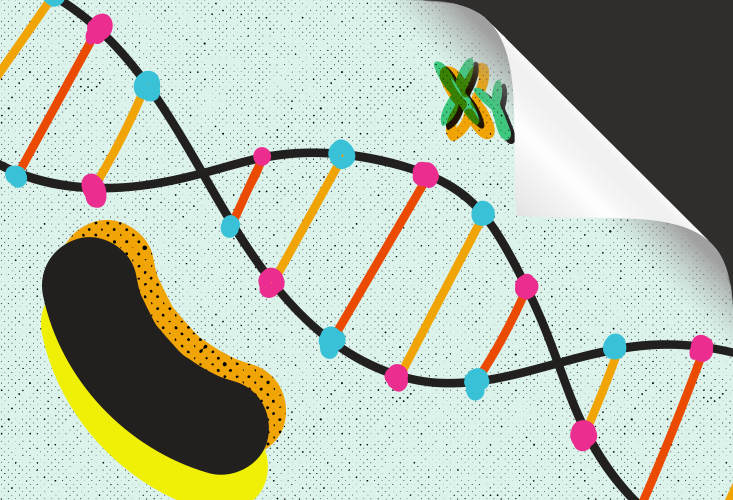
Some maternal and paternal effects appear to have evolved as a means to give offspring a head start in the type of environment that the offspring are likely to encounter.5 A classic example of such “anticipatory” parental effects is the induction of defenses in offspring of parents exposed to predators. “Water fleas” (Daphnia sp.) are tiny freshwater crustaceans that swim with a slow, jerking movement, using a pair of long appendages as oars. They are easy prey for predaceous insects, crustaceans, and fish. When they encounter chemical cues from predators, some species of Daphnia respond by growing spines on their head and tail, thus making themselves harder to grab or swallow. Daphnia exposed to predator cues also produce offspring that develop spines even in the absence of predator cues and may undergo changes in growth rate and life history that further reduce vulnerability to predation. Such trans-generational induction of anti-predator defenses also occurs in many plants; when attacked by herbivores such as caterpillars, plants produce seedlings that secrete unpalatable defensive chemicals (or are primed to initiate such defenses more rapidly in response to herbivore cues), and such induced defenses can persist for several generations.11-13
While it’s not yet known how Daphnia mothers induce spine development in their offspring, some examples of apparently adaptive maternal and paternal effects involve the transfer of particular compounds to offspring. For example, Utetheisa ornatrix moths obtain pyrrolizidine alkaloids by eating legumes that synthesize this toxin. Females are attracted to the scent of males that are well-endowed with this chemical, and such males transfer part of their toxic store as a “nuptial gift” in the seminal fluid. Females incorporate these alkaloids into their eggs, making their offspring unpalatable to predators.14,15
Stress may have deleterious effects not only on the individuals that experience it but also on their descendants.
Parents can also prime their offspring for the social environment and lifestyle that they are likely to encounter, as illustrated by the desert locust (Schistocerca gregaria). These insects can switch between two strikingly different phenotypic morphs: a green-gray “solitarious” morph, and a black-yellow “gregarious” morph. Gregarious locusts are characterized by lower fecundity, shorter lifespan, a larger brain, and a tendency to aggregate into enormous migratory swarms that can denude entire regions of vegetation. Locusts switch rapidly from solitary to gregarious behavior upon contact with a dense locust aggregation, and the population density experienced by females prior to mating also determines the morph of their offspring. Interestingly, however, the full suite of phenotypic changes builds up over several generations, indicating that this maternal effect is cumulative. The effect appears to be mediated by substances transmitted to the offspring via the egg cytoplasm and/or via accessory gland products that coat the eggs, although epigenetic modifications in the germ line could also play a role.16-20
However, parental experience does not necessarily prime offspring to perform better. For one thing, parents could misinterpret environmental cues, or the environment could change too rapidly, and this means that parents will sometimes adjust offspring traits for the wrong conditions. For example, if Daphnia mothers induce spine development in their offspring but predators then fail to materialize, the offspring will end up paying the costs of developing and bearing spines but reap no benefits from this trait. In such cases, anticipatory parental effects could actually harm offspring.21 More generally, offspring face the complex problem of integrating environmental cues received from their parents with cues received directly from their own environment, and their best developmental strategy will depend on which set of cues happens to be more useful and reliable.22
Anticipatory effects can misfire but, on the whole, such effects are still expected to be favored by natural selection. However, many parental effects are not adaptive in any sense at all. Stress may have deleterious effects not only on the individuals that experience it but also on their descendants. For example, research by Katie McGhee, Alison Bell, and colleagues at the University of Illinois showed that female sticklebacks that were exposed to mock predator attacks produced offspring that were slow learners, failed to behave appropriately when confronted with real predators, and were thus more likely to be eaten than offspring of naive mothers.23,24 These effects are reminiscent of the dire consequences of maternal smoking during pregnancy in our own species. Correlational studies on human cohorts (and experimental studies on rodents) show that, rather than priming the developing fetus for resistance to respiratory challenges, maternal smoking appears to alter the intrauterine environment in ways that predispose the child to reduced lung function and asthma, in addition to reduced birth weight, psychological disorders, and other problems.25-28 Similarly, in organisms ranging from yeast to humans, old parents tend to produce sickly or short-lived offspring. Although the transmission of genetic mutations via the germ line could contribute to such “parental age effects,” nongenetic inheritance appears to play a major role. Thus, although some types of parental effects represent evolved mechanisms that can enhance fitness, it is clear that some parental effects transmit pathology or stress. Such nonadaptive parental effects are comparable to deleterious genetic mutations, although they differ from genetic mutations in being consistently induced under particular conditions.
The fact that parental effects can sometimes be deleterious suggests that offspring should evolve ways to mitigate the harm, perhaps by blocking out certain types of nongenetic information received from their parents. This can occur even if the fitness interests of parents and their offspring are well aligned, since the transmission of erroneous environmental cues or parental pathology will be disadvantageous for both parents and their offspring. However, as Dustin Marshall, Tobias Uller, and others have noted, the fitness interests of parents and their offspring are rarely identical, and parental effects could therefore sometimes become an arena for parent-offspring conflict.5,29,30 Individuals are selected to allocate their resources in a way that maximizes their own fitness.31 Whenever an individual can expect to produce more than one offspring in its lifetime, it therefore faces a decision about how to carve up the pie among multiple progeny. For example, mothers might maximize their reproductive success by producing a greater number of offspring, even if this means investing less in each offspring.32 But, since each individual offspring could benefit by getting more resources from its mother, such “selfish” maternal strategies will be costly for offspring, and might select for counterstrategies that enable offspring to extract more resources from their mother.
To complicate matters even further, the interests of the mother and father might diverge as well. For example, as David Haig has pointed out, fathers might often benefit by helping their offspring to extract extra resources from their mother, even if this extra investment reduces the mother’s fitness. This is because, whenever males have an opportunity to sire offspring with multiple females, each of whom is also likely to mate with other males, a male’s best strategy is to selfishly exploit the resources of each of his mates for the benefit of his own offspring.33 Such parent-offspring and mother-father conflicts over parental investment are a potentially important but little-explored dimension of the evolution of nongenetic inheritance.
Of all the countless factors that compose an animal’s environment, diet stands out as particularly important for Darwinian fitness, health, and many other traits. Perhaps not surprisingly, it turns out that diet can also have major effects across generations. One of us has been studying the effects of diet in the beautiful neriid fly Telostylinus angusticollis, which breeds on rotting tree bark along the east coast of Australia. Neriid males are remarkably variable: In a typical aggregation on a tree trunk, one can spot 2-centimeter-long monsters alongside 5-millimeter-long dwarfs. Yet, when the flies are reared on a standard larval diet in the lab, all adult males turn out rather similar in size, indicating that much of the variation seen in the wild is environmental rather than genetic in origin; in other words, maggots lucky enough to encounter a nutrient-rich food patch develop into large adults, while those relegated to a nutrient-poor patch end up small.
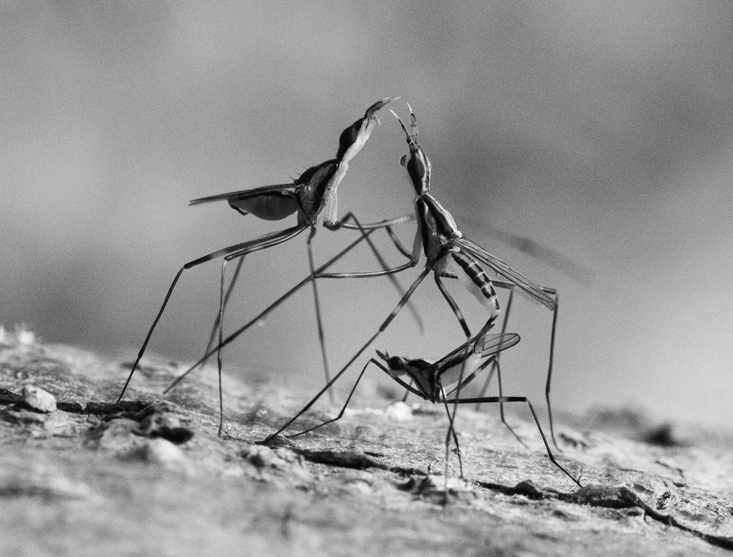
But is any of this enormous environmentally induced variation in male phenotype transmitted across generations? To find out, we generated variation in male body size by rearing some larvae on a nutrient-rich larval medium, while rearing their siblings on a diluted medium.
This resulted in sets of large and small brothers, which we then paired with females that had all been reared on the same larval food. When we measured the offspring, we found that large males produced larger offspring than their small brothers, and subsequent work showed that this nongenetic paternal effect is probably mediated by substances transferred in the seminal fluid.34,35 However, because T. angusticollis males transfer a tiny ejaculate, orders of magnitude smaller than the typical nutrient-laden ejaculates produced by males of some insect species, this effect does not appear to involve the transfer of nutrients from males to females or to their offspring.
We know just enough to fathom the depths of our ignorance and to recognize the challenges that lie ahead.
We have recently discovered that such effects can even extend to offspring sired by other males.35 Angela Crean produced large and small males as described earlier, and mated each female to both types of males. The first mating occurred while the female’s eggs were immature, but the second mating occurred two weeks later, after the eggs had matured and become encased in an impermeable shell. Females laid eggs soon after this second mating, and the offspring were collected and genotyped to determine paternity. Since fly eggs can only be fertilized when mature (with the sperm entering through a specialized opening in the eggshell), and females rarely store sperm for as long as two weeks, we were not surprised to see that almost all of the offspring had been sired by the males that mated second. Intriguingly, however, we found that the body size of the offspring was influenced by the larval diet of their mother’s first mate. In other words, offspring were larger when their mother first mated with a male that had been well fed as a maggot, even if this male was not their father. A separate experiment ruled out the possibility that females were adjusting their investment in eggs based on their visual or pheromonal assessment of the first male, leading us to conclude that molecules from the first male’s seminal fluid were absorbed by the female’s immature eggs (or, alternatively, somehow induced females to alter their investment in the developing eggs), and thereby affected the development of embryos sired by a different male. Such nonparental transgenerational effects (dubbed “telegony” by August Weismann) were widely discussed in the scientific literature before the advent of Mendelian genetics, but the early evidence was less than convincing. Our study provided the first modern confirmation that such effects are possible.36 Although telegony falls outside the scope of heredity in the usual sense of “vertical” (parent-offspring) transmission, it strikingly illustrates the potential for nongenetic inheritance to violate Mendelian assumptions.
There is ample evidence that parental diet can affect offspring in mammals as well. Experimental research on the effects of diet in rats—particularly the restriction of key nutrients such as protein—began in the first half of the 20th century with the objective of gaining insight into the health consequences of malnutrition. In the 1960s, researchers were intrigued to discover that female rats fed a low-protein diet during pregnancy produced offspring and grand-offspring that were sickly and scrawny and had relatively small brains with a reduced number of neurons, scoring poorly on tests of intelligence and memory. In recent years, research efforts have turned to understanding the effects of excessive or unbalanced nutrient intake, using rats and mice as experimental models to gain insight into the human obesity epidemic, and it is now well-established that both maternal and paternal diets can have a variety of effects on offspring development and health. Some of these effects come about via epigenetic reprogramming of embryonic stem cells in the womb. For example, in rats, a high-fat maternal diet has been shown to reduce the proliferation of hematopoietic stem cells (which give rise to blood cells), while a maternal diet rich in methyl-donors has been found to promote the proliferation of neuronal stem cells in embryos.37,38 In rats, a high-fat paternal diet has been found to cause reduced insulin secretion and glucose tolerance in daughters.39 There is evidence of such effects in humans as well.
Stepping back to survey the current state of knowledge in the study of extended heredity, we are reminded of genetics in the 1920s or molecular biology in the 1950s. We know just enough to fathom the depths of our ignorance and to recognize the challenges that lie ahead. But one conclusion that is already beyond reasonable doubt is that the Galtonian assumptions that have shaped both empirical and theoretical research for nearly a century are violated in many contexts, and this means that biology has exciting times before it. Empirical researchers will be busy for many years exploring the mechanisms of nongenetic inheritance, observing its ecological effects, and establishing its evolutionary consequences. This work will require developing new tools and devising ingenious experiments. Theoreticians have the equally important task of clarifying ideas and generating predictions. And on a practical level, in medicine and public health, it is now equally clear that we need not be “passive transmitters of a nature we have received,” because our life experiences play a nontrivial role in shaping the hereditary “nature” that we transmit to our children.
Russell Bonduriansky is professor of evolutionary biology at the University of New South Wales in Australia. Troy Day is a professor in the Department of Mathematics and Statistics and the Department of Biology at Queen’s University in Canada. His books include Biocalculus and A Biologist’s Guide to Mathematical Modeling in Ecology and Evolution.
Excerpted from Extended Heredity: A New Understanding of Inheritance and Evolution by Russell Bonduriansky and Troy Day. Copyright © 2018 by Princeton University Press. Reprinted by permission.
References
1. The “missing heritability” problem reflects the fact that genome-wide association studies have thus far failed to identify genes whose combined effects can account for the observed heritability of many traits, ranging from diseases that “run in the family” to strongly heritable traits such as human height. In other words, although relatives tend to exhibit similar phenotypes for these traits, relatives with similar phenotypes tend to have few genetic alleles in common, and it is therefore not clear what the trait’s genetic basis might be. Missing heritability could result from complex interactions between genes (epistasis), since such interactions are difficult to take into account in genome-wide association studies. Missing heritability could also arise if some of the heritable variation for the trait is nongenetic, especially if the nongenetic variation is induced by the environment.
2. Darwin, C.R. On the Origin of Species (1859).
3. Wolf, J.B. & Wade, M.J. What are maternal effects (and what are they not)? Philosophical Transactions of the Royal Society B 364, 1107-1115 (2009).
4. Badyaev, A.V. & Uller, T. Parental effects in ecology and evolution: mechanisms, processes, and implications. Philosophical Transactions of the Royal Society B 364, 1169-1177 (2009).
5. Marshall, D.J. & Uller, T. When is a maternal effect adaptive? Oikos 116, 1957-1963 (2007).
6. In evolutionary ecology, interest in maternal effects was spurred in part by the work of Tim Mousseau, Charles Fox, and others on seed beetles during the 1990s.
7. The presumed rarity of paternal effects is probably one reason why the “paternal half-sib” design became popular in quantitative genetics. While studies based on full siblings or maternal half-sibs could be compromised by common environment effects and maternal effects, it is often assumed that analysis of paternal half-sibs should yield accurate estimates of genetic (co)variance and heritability.
8. Crean, A.J. & Bonduriansky, R. What is a paternal effect? Trends in Ecology & Evolution 29, 554-559 (2014).
9. For an introduction to indirect genetic effects, see: Wolf, J.B., Brodie, E.D., Cheverud, J.M., Moore, A.J., & Wade, M.J. Evolutionary consequences of indirect genetic effects. Trends in Ecology & Evolution 13, 64-69 (1998).
10. Nelson, V.R., Spiezio, S.H. & Nadeau, J.H. Transgenerational genetic effects of the paternal Y chromosome on daughters’ phenotypes. Epigenomics 2, 513-521 (2010).
11. Agrawal, A.A., Laforsch, C., & Tollrian, R. Transgenerational induction of defences in animals and plants. Nature 401, 60-63 (1999).
12. Holeski, L.M., Jander, G. & Agrawal, A.A. Trans-generational defense induction and epigenetic inheritance in plants. Trends in Ecology & Evolution 27, 618-626 (2012).
13. Tolrian, R. Predator-induced morphological defences: costs, life history shifts, and maternal effects in Daphnia pulex. Ecology 76, 1691-1705 (1995).
14. Dussourd, D.E., et al. Biparental defensive endowment of eggs with acquired plant alkaloid in the moth Utetheisa ornatrix. Proceedings of the National Academy of Sciences 85, 5992-5996 (1988).
15. Smedley, S.R. & Eisener, T. Sodium: A male moth’s gift to its offspring. Proceedings of the National Academy of Sciences 93, 809-813 (1996).
16. Ernst, U.R., et al. Epigenetics and locust life phase transitions. Journal of Experimental Biology 218, 88-99 (2015).
17. Miller, G.A., Islam, M.S., Claridge, T.D.W., Dodgson, T., & Simpson, S.J. Swarm formation in the desert locust Schistocerca gregaria: Isolation and NMR analysis of the primary maternal gregarizing agent. Journal of Experimental Biology 211, 370-376 (2008).
18. Ott, S.R. & Rogers, S.M. Gregarious desert locusts have substantially larger brains with altered proportions compared with the solitarious phase. Proceedings of the Royal Society B 277, 3087-3096 (2010).
19. Simpson, S.J. & Miller, G.A. Maternal effects on phase characteristics in the desert locust, Schistocerca gregaria: A review of current understanding. Journal of Insect Physiology 53, 869-876 (2007).
20. Tanaka, S. & Maeno, K. A review of maternal and embryonic control of phase-dependent progeny characteristics in the desert locust. Journal of Insect Physiology 56, 911-918 (2010).
21. In fact, evidence that anticipatory effects typically increase fitness is equivocal. See: Uller, T., Nakagawa, S., & English, S. Weak evidence for anticipatory parental effects in plants and animals. Journal of Evolutionary Biology 26, 2161-2170 (2013).
22. Leimar, O. & McNamara, J.M. The evolution of transgenerational integration of information in heterogeneous environments. The American Naturalist 185, E55-69 (2015).
23. McGhee, K.E. & Bell, A.M. Paternal care in a fish: Epigenetics and fitness enhancing effects on offspring anxiety. Proceedings of the Royal Society B 281, E20141146 (2014).
24. McGhee, K.E., Pintor, L.M., Suhr, E.L., & Bell, A.M. Maternal exposure to predation risk decreases offspring antipredator behaviour and survival in three-spined stickleback. Functional Ecology 26, 932-940 (2012).
25. Hollams, E.M., de Klirk, N.H., Holt, P.G., & Sly, P.D. Persistent effects of maternal smoking during pregnancy on lung function and asthma in adolescents. American Journal of Respiratory and Critical Care Medicine 189, 401-407 (2014).
26. Knopik, V.S., Maccani, M.A., Francazio, S., & McGeary, J.E. The epigenetics of maternal cigarette smoking during pregnancy and effects on child development. Development and Psychopathy 24, 1377-1390 (2012).
27. Leslie, F.M. Multigenerational epigenetic effects of nicotine on lung function. BMC Medicine 11 (2013). Retrieved from DOI:10.1186/1741-7015-11-27.
28. Moylan, S., et al. The impact of maternal smoking during pregnancy on depressive and anxiety behaviors in children: The Norwegian mother and child cohort study. BMC Medicine 13 (2015). Retrieved from DOI:10.1186/s12916-014-0257-4.
29. Uller, T. & Pen, I. A theoretical model of the evolution of maternal effects under parent-offspring conflict. Evolution 65, 2075-2084 (2011).
30. Kuijper, B. & Johnstone, R.A. Maternal effects and parent-offspring conflict. Evolution 72, 220-233 (2018).
31. More precisely, natural selection favors strategies that maximize “inclusive fitness,” which reflects the fitness of the focal individual plus that of its relatives, with each relative’s contribution to the focal individual’s fitness weighted by its degree of relatedness (that is, its probability of sharing genetic alleles with the focal individual). This is why the brilliant and eccentric biologist J.B.S. Haldane once quipped that he would sacrifice his life for two brothers (each of whom shares 50 percent of his alleles, on average) or eight first cousins (each of whom shares 12.5 percent of his alleles, on average).
32. For the classic analysis of the problem of optimally trading off offspring quality against offspring number, see: Smith, C.C. & Fretwell, S.D. The optimal balance between size and number of offspring. The American Naturalist 108, 499-506 (1974).
33. Haig invoked genomic imprinting as the mechanism that males use to obtain a greater share of maternal resources for their offspring. Genomic imprinting involves differential methylation of alleles in eggs versus sperm, resulting in epigenetic marks that persist in the embryo and can differentially regulate expression of maternally and paternally inherited alleles. For example, expression of the Igf2 gene in the mammalian placenta regulates embryonic growth rate, and this gene is imprinted such that only the paternally inherited allele is expressed while the maternally inherited allele is silent. Haig suggested that imprinting of Igf2 originally evolved as a paternal strategy enabling offspring to extract a greater share of maternal resources and grow faster, with subsequent evolution of a maternal counter-strategy of shutting down expression of this gene, ultimately reaching a stalemate. However, males could evolve other mechanisms, such as paternal effects mediated by factors in the seminal fluid, to obtain a greater share of maternal resources for their offspring.
34. Bonduriansky, R. & Head, M. Maternal and paternal condition effects on offspring phenotype in Telostylinus angusticollis (Diptera: Neriidae). Journal of Evolutionary Biology 20, 2379-2388 (2007).
35. Crean, A.J. Kopps, A.M., & Bonduriansky, R. Revisiting telegony: Offspring inherit an acquired characteristic of their mother’s previous mate. Ecology Letters 17, 1545-1552 (2014).
36. A telegony-like effect has now also been reported in Drosophila. See: Garcia-Gonzalez, F. & Dowling, D.K. Transgenerational effects of sexual interactions and sexual conflict: non-sires boost the fecundity of females in the following generation. Biology Letters 11 (2015).
37. Kamimae-Lanning, A.N., et al. Maternal high-fat diet and obesity compromise fetal hematopoiesis. Molecular Metabolism 4, 25-38 (2015).
38. Amarger, V., et al. Protein content and methyl donors in maternal diet interact to influence the proliferation rate and cell fate of neural stem cells in rat hippocampus. Nutrients 6, 4200-4217 (2014).
39. Ng, S.F., et al. Chronic high-fat diet in fathers programs β-cell dysfunction in female rat offspring. Nature 467, 963-966 (2010).