An 86-year-old patient arrives with a grisly foot injury.1 It’s badly infected—not a surprise, given his chronic untreated Type 2 diabetes. What is surprising is that meropenem, a broad spectrum antibiotic, and vancomycin, known as the antibiotic of last resort, have absolutely no effect.
The doctors know something bad is going on. But, even expecting the worst, the test results surprise them. The man’s foot is infected with not one, but three different bacteria: Staphylococcus aureus, Acinetobacter baumannii, and Acinetobacter lwoffii. Each is multi-drug resistant. The hospital, located in Brazil, simply doesn’t have the resources to deal with the situation. The patient is transferred to a larger hospital, but enough damage has already been done to his foot to require amputation.
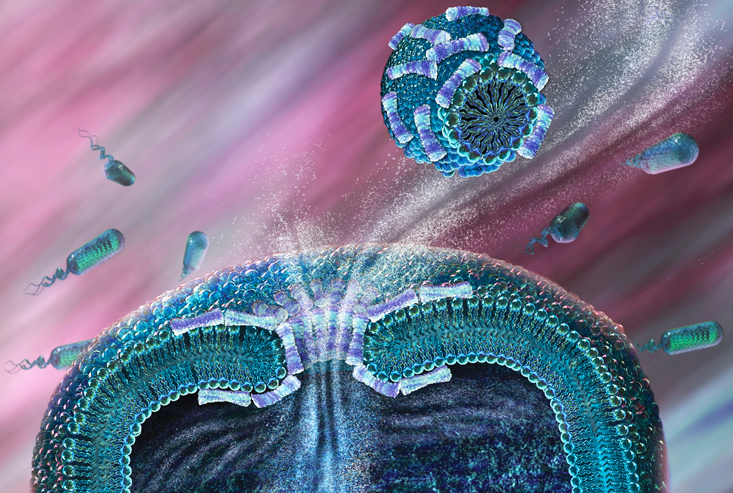
This real story, reported in 2012, is one of many. There was also the 57-year old woman in Washington, D.C. whose heart failure was caused by a penicillin-resistant bacteria.2 Or the woman who died in quarantine after being admitted to a Nevada hospital with an infection resistant to every antibiotic the hospital had access to.3
An estimated 2 million Americans are infected with antibiotic resistant microbes every year and, of these, about 23,000 die.4 Humans have known how to kill bacteria since before the dawn of civilization. So why is killing bacteria in patients resisting the combined efforts of modern science?
The first steps to antibiotic therapy were made by the British surgeon Joseph Lister in 1867.5 Lister noticed that many of his surgical patients required amputations or died shortly after their procedures. Many credited this to the influence of “miasmas” (noxious, “bad” air) or oxygen on the open wound.
Lister had a different theory. He had been following the work of French microbiologist Louis Pasteur, who had shown that the spoilage of food was not due to oxygen, but to tiny, microscopic organisms. Lister hypothesized that these same organisms were responsible for his patients’ terrible outcomes. Pasteur had given three options for avoiding this outcome: filtering the organisms away, cooking them to death, or killing them with a chemical. The first two options could be immediately ruled out, but the third proved intriguing.
Would it be possible to make a chemical magic bullet that would kill bacteria but not human cells?
Lister, being a curious and scholarly scientist, had recently heard of the use of creosote, or coal tar distillate, to prevent railway ties from rotting. Intuiting that these same troublesome microorganisms were to blame, he decided to try a component of coal tar distillate, carbolic acid, to treat his patients’ wounds. The initial results were striking: Patients with compound fractures, who almost universally required amputation, were now able to make full recoveries with their limbs intact.
What Lister had found was the first medical antiseptic—not an antibiotic. Carbolic acid was poisonous to humans too, so it could only be applied sparingly to wounds. Paul Ehrlich, a prolific and brilliant German scientist, wanted to do better. He was fascinated with the old folktale of the Freischütz, a marksman who made a deal with the devil to receive six magic bullets that would hit a target and avoid everything that might get in the way. Would it be possible to make a chemical magic bullet that would kill bacteria but not human cells?
Ehrlich’s background was in histology, particularly in staining samples for viewing under the microscope. Ehrlich discovered that certain dye molecules would stain some cells but not others, seeking out their targets like the mythical magic bullets. Ehrlich theorized that the right dye molecules might be able to realize his dream of a selective antibiotic.
Finally, in 1909, Ehrlich’s idea was achieved in arsphenamine, an arsenic-based dye able to kill syphilis bacteria without killing the patient. Arsphenamine was only really effective against syphilis, though. Researchers at the Bayer sector of the German conglomerate, IG Farben, wondered if this same approach could lead to an antibiotic for broader use. Chemists Josef Klarer and Fritz Miestzsch synthesized thousands of dyes that were tested on infected laboratory mice by Gerhard Domagk. After countless failures, one dye, prontosil, succeeded, delivering the first truly versatile antibiotic.
While the Bayer team assumed that Ehrlich’s dye hypothesis was the reason for prontosil’s effectiveness, subsequent research showed that it has nothing to do with its ability to stain cells.
So what makes an antibiotic tick?
It’s relatively easy to find new antiseptics, which can kill microbes on the skin and the surface of tissues. But antiseptics make terrible antibiotics: Chemicals that break an essential part of a bacterial cell will usually break the same part of human cells. Luckily, sometimes there are bacterial parts not found in humans, or if they are, they are very different. This is the key to antibiotic function: exploiting the fact that bacteria are similar to human cells without being identical.
In the century or so since the first antibiotic was found, we’ve discovered a small library of bacteria-specific features to disrupt.6 The antibiotic sulfanilamide, for example, targets a part of bacterial life that doesn’t exist in humans. Folates like vitamin B9 are essential for DNA synthesis in all organisms. Humans get them by eating fruits and vegetables, but bacteria have to make them from scratch through processes foreign to human cells. Like other sulfa drugs, sulfanilamide plugs up one enzyme in one pathway of one process, stopping bacterial DNA synthesis in its tracks while having no effects on human metabolism.
Penicillin, serendipitously discovered by Alexander Fleming in 1928, and other beta lactam antibiotics (like meropenem) also target a part of bacteria that human cells don’t have: the cell wall. Bacterial cells are a lot like an overstuffed roast being kept together by butcher’s twine. When that butcher’s twine—the cell wall—is removed, bacterial cells literally explode.
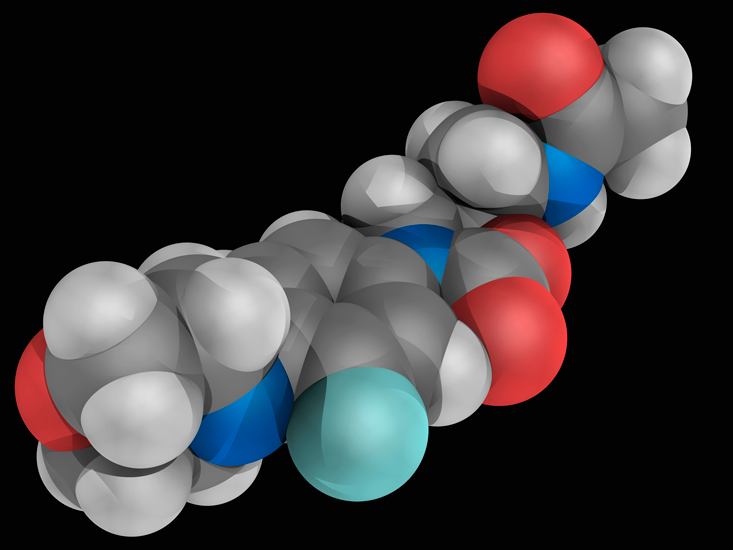
Bacteria build their cell walls a lot like how people build fences. After putting up some fence posts, the horizontal supports and boards are attached with the equivalent of a nail gun. What penicillin does is jam the bacteria’s nail gun, preventing any of the posts in its cell wall from being connected. Glycopeptide antibiotics like vancomycin, on the other hand, wrap around the cell wall’s fence posts like a thick bulletproof blanket. The nail gun still works, but none of the nails can get into the fence post.
These are the ideal cases. The other main classes of antibiotics target parts of bacterial life that are more similar to mechanisms in human cells—but still just different enough to allow them to be targeted. These differences can be very subtle, with the active walking a fine line between being selective antibiotics and antiseptics.
Many drugs target the manufacture of proteins, for example. Antibiotics can prevent tightly wound DNA from being unpacked and read, jam the RNA transcription process, or shut down the molecular factory that turns molecular RNA into proteins. In each case, the equivalent process in a human cell is performed by enzymes that are shaped differently and don’t have the same handholds that the antibiotics need to do their work. It would be a problem if they did, however, as stopping any of these activities is just as deadly for human cells as for bacteria.
That’s the offense side of the story. There is also the defense: Bacteria have a tendency to fight back. One simple defense is to simply kick the antibiotics out before they can cause any damage. Like how a sump pump constantly removes water from a basement to keep it from flooding, bacterial efflux pumps constantly remove antibiotics to keep them from doing their work. One efflux pump might give multi-drug resistance by recognizing and removing several different kinds of antibiotics, making this a difficult resistance mechanism to deal with.
Bacteria can also make new proteins that break open and disarm antibiotics before they can act. Perhaps the most well-known example of this strategy is the bacterial manufacture of penicillinase, a new enzyme whose sole function is to break open the spring-loaded penicillin molecule before it can gum up the machinery that makes cell walls. These types of proteins are quite specific to one kind of antibiotic and don’t usually give resistance to other classes. One way that we’ve been able to defeat this resistance mechanism is to package the original antibiotic with a new one for the new enzyme.
Chemicals that break an essential part of a bacterial cell will usually break the same part of human cells.
Another defense bacteria have is to make proteins that fit antibiotics with molecular straightjackets, preventing them from grabbing onto their targets and rendering them helpless bystanders. These enzymes work by attaching chemical groups known as phosphoryl, acetyl, nucleotidyl, glycosyl, or hydroxyl to key parts of the antibiotics, blocking them from interacting with the part of the bacteria they’re supposed to disable. These enzymes are usually selective for members of one antibiotic family, so, again, cross resistance is often not a problem.
Perhaps the most obvious and confounding method of resistance bacteria have at their disposal is simply changing the target of an antibiotic so much that it no longer recognizes it. This method of resistance is very common, and there are many ways to accomplish it. For example, just changing the end-cap of the cell wall posts from the amino acid D-alanine to D-lactate, a very small adjustment, makes aminoglycoside antibiotics like vancomycin completely useless. Once the target has changed, it’s impossible to hit it with the same magic bullet.
In principle, the response to this kind of resistance is to simply find a new magic bullet. But many antibiotics have come from microorganisms themselves, where the fight for survival has forced one species to make weapons to defeat their competitors. The way these microorganisms typically make antibiotics is not very flexible: They are very good at making a specific key but have trouble adjusting it if it stops fitting into the target’s lock. We have also discovered many of the obvious antibiotics derived from nature, and are running out of low-hanging fruit. All but one (ceftaroline) of the broad-spectrum antibiotics we use today were discovered over a decade ago, with almost half found in the “Golden Age” between 1950 and 1960.7
There are many other approaches. One that is attracting a lot of attention is the pursuit of cryptic antibiotics, which involves forcing bacteria to make molecules that they don’t usually make.8 The jury is still out on whether this approach will work. Our best option today is often to turn to organic chemistry, which has given us exquisite tools to tune molecules in ways otherwise unimaginable.9 Linezolid, an antibiotic that stops the ribosome from laying the foundation for new proteins, was invented from scratch by human beings using the techniques of organic chemistry. We can also use organic chemistry to tweak antibiotics that we discover in nature. The company Tetraphase, for example, is working to adjust tetracyclines to fit a variety of resistant bacterial locks.
The one thing we can’t do is give up, because bacteria never will. Pharmaceuticals have spent billions searching for new antibiotics, though many gave up after finding nothing but failure.10 This is dangerous. We need to understand that the search will be slow and difficult. I’ve spent my career designing better organic chemistry tools for making molecules like antibiotics faster, but even with these new inventions, the process isn’t easy. Antibiotic resistance is an arms race where humans are starting to fall behind. Unless we want to lose, we need to do more now. All our lives depend on it.
Julian G. West is a chemist and writer in Pasadena, CA. He is currently a postdoctoral fellow at the California Institute of Technology. Follow him on Twitter @pushingarrows.
References
1. Neto, R.M., Ansaldi Jr., M.A., da Costa, M.E.S.M., da Silva, S.M., & Luz, V.H.F. Report of a multi-drug resistant bacterial infection in a diabetic patient treated in northeast Brazil. Diabetic Foot & Ankle 3, 18656 (2012).
2. Siegel, M. & Timpone, J. Penicillin-resistant Streptococcus pnuemoniae endocarditis: A case report and review. Clinical Infectious Diseases 32, 972–974 (2001).
3. Chen, L., Todd, R., Kiehlbauch, J., Walters, M., & Kallen, A. Pan-resistant New Delhi metallo-beta-lactamase-producing Klebsiella pneumonia—Washoe County, Nevada, 2016. Morbidity and Mortality Weekly Report 66, 33 (2017).
4. Centers for Disease Control and Prevention. Antibiotic Resistance Threats in the United States, 2013. Retrieved from https://www.cdc.gov/drugresistance/threat-report-2013/index.html
5. Rosen, W. Miracle Cure: The Creation of Antibiotics and the Birth of Modern Medicine Viking, New York, NY (2017).
6. Kapoor, G., Saigal, S., & Elongavan, A. Action and resistance mechanisms of antibiotics: A guide for clinicians. Journal of Anaesthesiology Clinical Pharmacology 33, 300–305 (2017).
7. Davis, J. Where have all the antibiotics gone? Canadian Journal of Infectious Diseases and Medical Microbiology 17, 287–290 (2006).
8. Rosen, P.C. & Seyedsayamdost, M.R. Though much is taken, much abides: Finding new antibiotics using old ones. Biochemistry 56, 4925–4926 (2017).
9. Liu, J., Bedell, T.A., West, J.G., & Sorensen, E.J. Design and synthesis of molecular scaffolds with anti-infective activity. Tetrahedron 72, 3579–3592 (2016).
10. Lowe, D. Antibiotics: Not As Easy As They Say. http://blogs.sciencemag.org (2015).