Before there is life, there must be structure. Our universe synthesized atomic nuclei early in its history. Those nuclei ensnared electrons to form atoms. Those atoms agglomerated into galaxies, stars, and planets. At last, living things had places to call home. We take it for granted that the laws of physics allow for the formation of such structures, but that needn’t have been the case.
Over the past several decades, many scientists have argued that, had the laws of physics been even slightly different, the cosmos would have been devoid of complex structures. In parallel, cosmologists have come to realize that our universe may be only one component of the multiverse, a vast collection of universes that makes up a much larger region of spacetime. The existence of other universes provides an appealing explanation for the apparent fine-tuning of the laws of physics. These laws vary from universe to universe, and we live in a universe that allows for observers because we couldn’t live anywhere else.
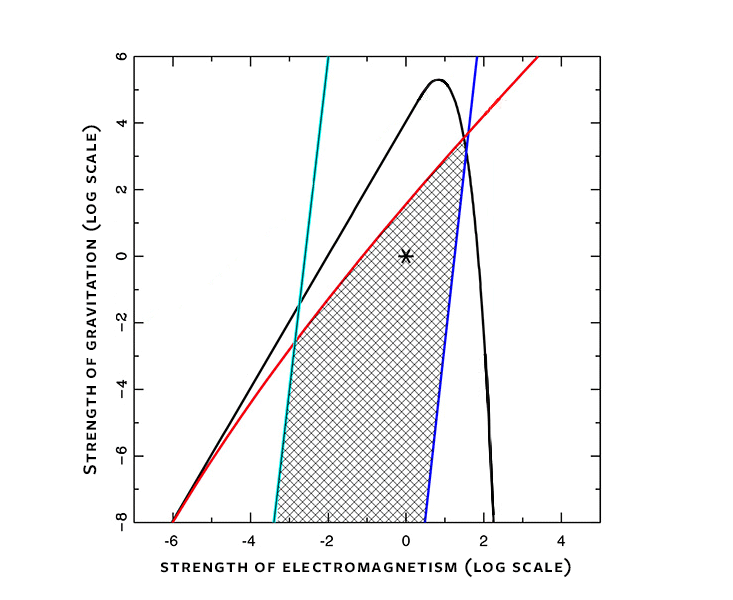
Astrophysicists have discussed fine-tuning so much that many people take it as a given that our universe is preternaturally fit for complex structures. Even skeptics of the multiverse accept fine-tuning; they simply think it must have some other explanation. But in fact the fine-tuning has never been rigorously demonstrated. We do not really know what laws of physics are necessary for the development of astrophysical structures, which are in turn necessary for the development of life. Recent work on stellar evolution, nuclear astrophysics, and structure formation suggest that the case for fine-tuning is less compelling than previously thought. A wide variety of possible universes could support life. Our universe is not as special as it might seem.
The first type of fine-tuning involves the strengths of the fundamental forces of nature in working stars. If the electromagnetic force had been too strong, the electrical repulsion of protons would shut down nuclear fusion in stellar cores, and stars would fail to shine. If electromagnetism had been too weak, nuclear reactions would run out of control, and stars would blow up in spectacular explosions. If gravity had been too strong, stars would either collapse into black holes or never ignite.
On closer examination, though, stars are remarkably robust. The strength of the electric force could vary by a factor of nearly 100 in either direction before stellar operations would be compromised. The force of gravity would have to be 100,000 times stronger. Going in the other direction, gravity could be a billion times weaker and still allow for working stars. The allowed strengths for the gravitational and electromagnetic forces depend on the nuclear reaction rate, which in turn depends on the strengths of the nuclear forces. If the reaction rate were faster, stars could function over an even wider range of strengths for gravitation and electromagnetism. Slower nuclear reactions would narrow the range.
Throughout much of the galaxy, the night sky could have the same brightness as the sunshine we see during the day on Earth.
In addition to these minimal operational requirements, stars must meet a number of other constraints that further restrict the allowed strength of the forces. They must be hot. The surface temperature of a star must be high enough to drive the chemical reactions necessary for life. In our universe, there are ample regions around most stars where planets are warm enough, about 300 kelvins, to support biology. In universes where the electromagnetic force is stronger, stars are cooler, making them less hospitable.
Stars must also have long lives. The evolution of complex life forms takes place over enormous spans of time. Since life is driven by a complex ensemble of chemical reactions, the basic clock for biological evolution is set by the time scales of atoms. In other universes, these atomic clocks will tick at different rates, depending on the strength of electromagnetism, and this variation must be taken into account. When the force is weaker, stars burn their nuclear fuel faster, and their lifetimes decrease.
Finally, stars must be able to form in the first place. In order for galaxies and, later, stars to condense out of primordial gas, the gas must be able to lose energy and cool down. The cooling rate depends (yet again) on the strength of electromagnetism. If this force is too weak, gas cannot cool down fast enough and would remain diffuse instead of condensing into galaxies. Stars must also be smaller than their host galaxies—otherwise star formation would be problematic. These effects put another lower limit on the strength of electromagnetism.
Putting it all together, the strengths of the fundamental forces can vary by several orders of magnitude and still allow planets and stars to satisfy all the constraints (as illustrated in the figure below). The forces are not nearly as finely tuned as many scientists think.
A second example of possible fine-tuning arises in the context of carbon production. After moderately large stars have fused the hydrogen in their central cores into helium, helium itself becomes the fuel. Through a complicated set of reactions, helium is burned into carbon and oxygen. Because of their important role in nuclear physics, helium nuclei are given a special name: alpha particles. The most common nuclei are composed of one, three, four, and five alpha particles. The nucleus with two alpha particles, beryllium-8, is conspicuously absent, and for a good reason: It is unstable in our universe.
The instability of beryllium creates a serious bottleneck for the creation of carbon. As stars fuse helium nuclei together to become beryllium, the beryllium nuclei almost immediately decay back into their constituent parts. At any given time, the stellar core maintains a small but transient population of beryllium. These rare beryllium nuclei can interact with helium to produce carbon. Because the process ultimately involves three helium nuclei, it is called the triple-alpha reaction. But the reaction is too slow to produce the amount of carbon observed in our universe.
To resolve this discrepancy, physicist Fred Hoyle predicted in 1953 that the carbon nucleus has to have a resonant state at a specific energy, as if it were a little bell that rang with a certain tone. Because of this resonance, the reaction rates for carbon production are much larger than they would be otherwise—large enough to explain the abundance of carbon found in our universe. The resonance was later measured in the laboratory at the predicted energy level.
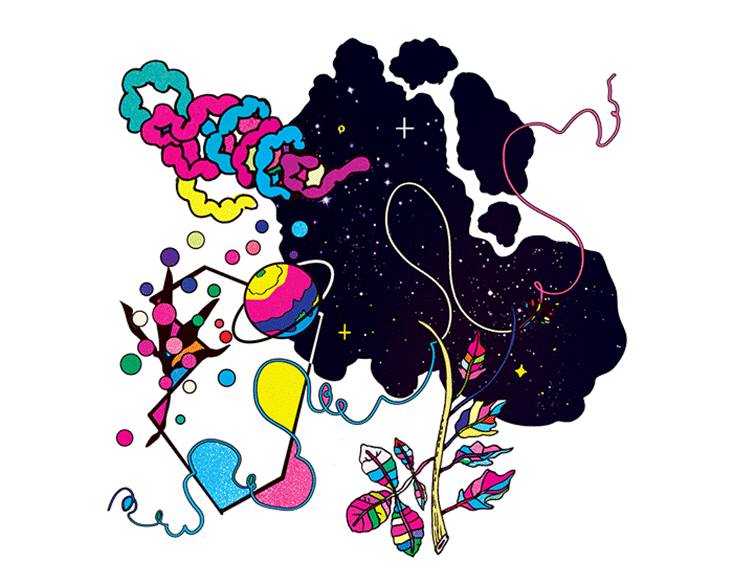
The worry is that, in other universes, with alternate strengths of the forces, the energy of this resonance could be different, and stars would not produce enough carbon. Carbon production is compromised if the energy level is changed by more than about 4 percent. This issue is sometimes called the triple-alpha fine-tuning problem.
Fortunately, this problem has a simple solution. What nuclear physics takes away, it also gives. Suppose nuclear physics did change by enough to neutralize the carbon resonance. Among the possible changes of this magnitude, about half would have the side effect of making beryllium stable, so the loss of the resonance would become irrelevant. In such alternate universes, carbon would be produced in the more logical manner of adding together alpha particles one at a time. Helium could fuse into beryllium, which could then react with additional alpha particles to make carbon. There is no fine-tuning problem after all.
A third instance of potential fine-tuning concerns the simplest nuclei composed of two particles: deuterium nuclei, which contain one proton and one neutron; diprotons, consisting of two protons; and dineutrons, consisting of two neutrons. In our universe, only deuterium is stable. The production of helium takes place by first combining two protons into deuterium.
If the strong nuclear force had been even stronger, diprotons could have been stable. In this case, stars could have generated energy through the simplest and fastest of nuclear reactions, where protons combine to become diprotons and eventually other helium isotopes. It is sometimes claimed that stars would then burn through their nuclear fuel at catastrophic rates, resulting in lifetimes that are too short to support biospheres. Conversely, if the strong force had been weaker, then deuterium would be unstable, and the usual stepping stone on the pathway to heavy elements would not be available. Many scientists have speculated that the absence of stable deuterium would lead to a universe with no heavy elements at all and that such a universe would be devoid of complexity and life.
As it turns out, stars are remarkably stable entities. Their structure adjusts automatically to burn nuclear fuel at exactly the right rate required to support themselves against the crush of their own gravity. If the nuclear reaction rates are higher, stars will burn their nuclear fuel at a lower central temperature, but otherwise they will not be so different. In fact, our universe has an example of this type of behavior. Deuterium nuclei can combine with protons to form helium nuclei through the action of the strong force. The cross section for this reaction, which quantifies the probability of its occurrence, is quadrillions of times larger than for ordinary hydrogen fusion. Nonetheless, stars in our universe burn their deuterium in a relatively uneventful manner. The stellar core has an operating temperature of 1 million kelvins, compared to the 15 million kelvins required to burn hydrogen under ordinary conditions. These deuterium-burning stars have cooler centers and are somewhat larger than the sun, but are otherwise unremarkable.
A wide variety of possible universes could support life. Our universe is not as special as it might seem.
Similarly, if the strong nuclear force were lower, stars could continue to operate in the absence of stable deuterium. A number of different processes provide paths by which stars can generate energy and synthesize heavy elements. During the first part of their lives, stars slowly contract, their central cores grow hotter and denser, and they glow with the power output of the sun. Stars in our universe eventually become hot and dense enough to ignite nuclear fusion, but in alternative universes they could continue this contraction phase and generate power by losing gravitational potential energy. The longest-lived stars could shine with a power output roughly comparable to the sun for up to 1 billion years, perhaps long enough for biological evolution to take place.
For sufficiently massive stars, the contraction would accelerate and become a catastrophic collapse. These stellar bodies would basically go supernova. Their central temperatures and densities would increase to such large values that nuclear reactions would ignite. Many types of nuclear reactions would take place in the death throes of these stars. This process of explosive nucleosynthesis could supply the universe with heavy nuclei, in spite of the lack of deuterium.
Once such a universe produces trace amounts of heavy elements, later generations of stars have yet another option for nuclear burning. This process, called the carbon-nitrogen-oxygen cycle, does not require deuterium as an intermediate state. Instead, carbon acts as a catalyst to instigate the production of helium. This cycle operates in the interior of the sun and provides a small fraction of its total power. In the absence of stable deuterium, the carbon-nitrogen-oxygen cycle would dominate the energy generation. And this does not exhaust the options for nuclear power generation. Stars could also produce helium through a triple-nucleon process that is roughly analogous to the triple-alpha process for carbon production. Stars thus have many channels for providing both energy and complex nuclei in alternate universes.
A fourth example of fine-tuning concerns the formation of galaxies and other large-scale structures. They were seeded by small density fluctuations produced in the earliest moments of cosmic time. After the universe had cooled down enough, these fluctuations started to grow stronger under the force of gravity, and denser regions eventually become galaxies and galaxy clusters. The fluctuations started with a small amplitude, denoted Q, equal to 0.00001. The primeval universe was thus incredibly smooth: The density, temperature, and pressure of the densest regions and of the most rarefied regions were the same to within a few parts per 100,000. The value of Q represents another possible instance of fine-tuning in the universe.
If Q had been lower, it would have taken longer for fluctuations to grow strong enough to become cosmic structures, and galaxies would have had lower densities. If the density of a galaxy is too low, the gas in the galaxy is unable to cool. It might not ever condense into galactic disks or coalesce into stars. Low-density galaxies are not viable habitats for life. Worse, a long enough delay might have prevented galaxies from forming at all. Beginning about 4 billion years ago, the expansion of the universe began to accelerate and pull matter apart faster than it could agglomerate—a change of pace that is usually attributed to a mysterious dark energy. If Q had been too small, it could have taken so long for galaxies to collapse that the acceleration would have started before structure formation was complete, and further growth would have been suppressed. The universe could have ended up devoid of complexity, and lifeless. In order to avoid this fate, the value of Q cannot be smaller by more than a factor of 10.
What if Q had been larger? Galaxies would have formed earlier and ended up denser. That, too, would have posed a danger for the prospects of habitability. Stars would have been much closer to one another and interacted more often. In so doing, they could have stripped planets out of their orbits and sent them hurtling into deep space. Furthermore, because stars would be closer together, the night sky would be brighter—perhaps as bright as day. If the stellar background were too dense, the combined starlight could boil the oceans of any otherwise suitable planets.
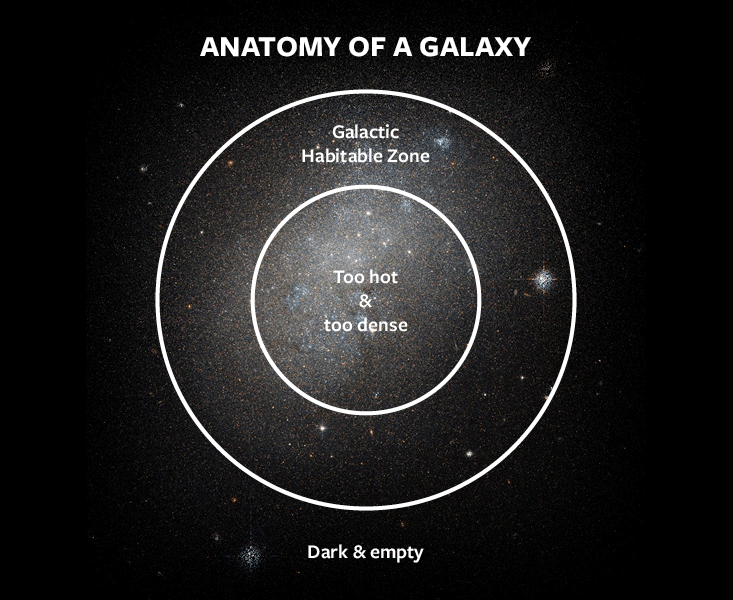
In this case, the fine-tuning argument is not very constraining. The central regions of galaxies could indeed produce such intense background radiation that all planets would be rendered uninhabitable. But the outskirts of galaxies would always have a low enough density for habitable planets to survive. An appreciable fraction of galactic real estate remains viable even when Q is thousands of times larger than in our universe. In some cases, a galaxy might be even more hospitable. Throughout much of the galaxy, the night sky could have the same brightness as the sunshine we see during the day on Earth. Planets would receive their life-giving energy from the entire ensemble of background stars rather than from just their own sun. They could reside in almost any orbit. In an alternate universe with larger density fluctuations than our own, even Pluto would get as much daylight as Miami. As a result, a moderately dense galaxy could have more habitable planets than the Milky Way.
In short, the parameters of our universe could have varied by large factors and still allowed for working stars and potentially habitable planets. The force of gravity could have been 1,000 times stronger or 1 billion times weaker, and stars would still function as long-lived nuclear burning engines. The electromagnetic force could have been stronger or weaker by factors of 100. Nuclear reaction rates could have varied over many orders of magnitude. Alternative stellar physics could have produced the heavy elements that make up the basic raw material for planets and people. Clearly, the parameters that determine stellar structure and evolution are not overly fine-tuned.
Given that our universe does not seem to be particularly fine-tuned, can we still say that our universe is the best one for life to develop? Our current understanding suggests that the answer is no. One can readily envision a universe that is friendlier to life and perhaps more logical. A universe with stronger initial density fluctuations would make denser galaxies, which could support more habitable planets than our own. A universe with stable beryllium would have straightforward channels available for carbon production and would not need the complication of the triple-alpha process. Although these issues are still being explored, we can already say that universes have many pathways for the development of complexity and biology, and some could be even more favorable for life than our own. In light of these generalizations, astrophysicists need to reexamine the possible implications of the multiverse, including the degree of fine-tuning in our universe.
Fred Adams is a physics professor at the University of Michigan in Ann Arbor. He is the recipient of the Helen B. Warner Prize from the American Astronomical Society, the National Science Foundation Young Investigator Award, and numerous teaching awards from the University of Michigan. He is co-author of The Five Ages of the Universe: Inside the Physics of Eternity and of Origins of Existence: How Life Emerged in the Universe.
This article was originally published on Nautilus Cosmos in January 2017.