How about that! Mr. Galileo was correct in his findings.” That conclusion wasn’t based on the most careful experiment you’ll ever see, but it was one of the most spectacular in its way—because it was performed on the moon.
In 1971, Apollo 15 astronaut David Scott dropped a feather and a hammer from the same height and found that they hit the lunar surface at the same time. The acceleration due to gravity doesn’t depend on a body’s mass or composition, just as Galileo asserted from his (probably apocryphal) experiment on the Leaning Tower of Pisa.
Or does it? Jump forward to the front-page headline of The New York Times in January 1986: “Hints of 5th Force in the Universe Challenge Galileo’s Findings.” The newspaper was reporting on a paper in the premier physics journal Physical Review Letters by physicist Ephraim Fischbach and his colleagues, describing evidence that the acceleration due to gravity does vary depending on the chemical composition of the object in question. Gravity, it seemed, was not quite what we thought it was: its effects are modified by what the The New York Times’ reporter John Noble Wilford christened a “fifth force,” adding to the four fundamental forces we already know.
More than 30 years later, many experiments have sought to verify this putative fifth force. Yet despite their extraordinary accuracy, none has ever found convincing evidence for it. That search shows no sign of abating, however. Even in the past year a new tantalizing hint that such a force exists has emerged from experiments in nuclear physics, provoking fresh speculation and excitement.
What hangs in the balance are some of the foundational principles of modern physics. Some physicists believe that a fifth force is permitted, even demanded, by efforts to extend and unify the current fundamental theories. Others hope such a force might shed light on the mysterious dark matter that seems to outweigh all the ordinary matter in the universe. If it exists, says physicist Jonathan Feng of the University of California, Irvine, “it would imply that our attempts to unify the known forces have been premature, as now there will be a fifth one to unify, too.”
Why speculate about another fundamental force of nature, when there’s no good evidence for it? The original motivation was appreciated even in Galileo’s time: There are two ways of thinking about mass. One comes from inertia: An object’s mass is its “resistance” to being moved, this being greater the more massive it is. The other comes from gravity: According to Isaac Newton’s law of universal gravitation, the force of gravity experienced between two masses, such as an apple and the Earth, is proportional to the product of their masses divided by the square of the distance between them. This force causes a falling apple to accelerate. If, and only if, the two definitions of mass are the same, the gravitational acceleration doesn’t depend on the amount of mass being accelerated.
Are they the same, though? If they aren’t, then different masses would fall under gravity at different rates. The intuitive notion that a greater mass should “fall faster” had motivated tests before Galileo. The Dutch natural philosopher Simon Stevin is thought to have dropped lead balls from the clock tower in Delft around 1586, finding no detectable difference in how long they took to reach the ground. Newton himself tested the idea around 1680 by measuring whether pendulums of different mass but identical length have the same period of swing—as they should if gravitational acceleration is mass-independent. His studies were repeated with more accuracy by the German scientist Friedrich Wilhelm Bessel in 1832. Neither of them found any detectable difference.
Gravity might be fine as it stands—but there might be a new, fifth force that makes it look different.
The idea that inertial and gravitational mass are the same is known as the weak equivalence principle. It became a crucial issue when Einstein formulated his theory of general relativity around 1912-16, which rested on the central idea that the acceleration caused by gravity is the same as the acceleration of an object subject to the same force in free space. If that’s not true, general relativity won’t work.
“The equivalence principle is one of the basic assumptions of general relativity,” says Stephan Schlamminger, who works at the Mecca of high-precision measurement, the National Institute of Standards and Technology in Gaithersburg, Maryland. “As such, it should be thoroughly tested. Tests of the equivalence principle are relatively cheap and simple, but could have a huge impact if a violation was found. It would be careless not to perform these experiments.”
If the weak equivalence principle fails, then there are two possibilities. Either Newton’s expression for the force of gravity between two masses (which is also what general relativity predicts if gravity is not extreme) is slightly inaccurate and needs tweaking. Or gravity might be fine as it stands—but there might be a new, fifth force that makes it look different. That fifth force would add to the four we already know to exist: gravity, electromagnetism, and the strong and weak nuclear forces that govern the interactions of subatomic particles inside atomic nuclei. Whether we think about “modified gravity” or a fifth force is, says Fischbach, in the end just a semantic distinction.
Either way, says Feng, there is “no reason at all that there can’t be a fifth force that we have not noticed until now.”
By the time Einstein pinned his new gravitational theory to it, the weak equivalence principle had already undergone some very exacting tests. At the end of the 19th century a Hungarian nobleman named Baron Loránd Eőtvős, working at the University of Budapest, realized it could be tested by placing two masses in delicate balance.
Eőtvős used an instrument known as a torsion balance. He attached two objects to the ends of a horizontal rod suspended by a thread. If the objects have the same weight—the same gravitational mass—then the rod is balanced horizontally. But the masses also experience a centrifugal force due to the rotation of the Earth, which depends on the objects’ inertial masses. If inertial mass is the same as the gravitational mass, all the forces are in balance and the rod stays still. But if they differ, then the masses will tend to swing away from the horizontal because of the Earth’s rotation.
And if the two masses experience a different “swing”—one possibility would be because the deviation from the weak equivalence principle is dependent on composition—then the rod will experience a net twisting force (torque), and it will rotate. Even if this rotation is very slight, it might be detected by, say, measuring the deflection of a light beam from a mirror attached to the rod.
Now, the fact is that the force of gravity does vary slightly from place to place on the Earth anyway. That’s because the planet is not a smooth uniform sphere. Rocks have different density, and so exert a very slightly different gravitational tug. And at the precision of Eőtvős’s experiments, even the presence of the nearby university buildings could disturb the results. One way of eliminating these local variations is to carry out the measurements for two different orientations of the dangling rod—say, east-west and north-south. Both should experience the same local effects of gravity, but the centrifugal forces will differ—and thus any deviation from weak equivalence would show up as a difference in torque between the two measurements. This approach fits with the general strategy of setting up the balance experiment to be sensitive to differences in gravitational acceleration between two test masses or configurations: That way, you don’t need to worry about local effects or about how accurately you can measure absolute forces.
Local perturbations might, however, also vary in time: Even a passing truck could induce a tiny gravitational disturbance. So the researchers had to take care to rule out such things. In fact, even the presence of the observing experimenter might matter. So the Hungarian scientists would stand well off as the balance came to rest, then dash into the lab to make a measurement before it had time to adjust to their presence (its twisting period was a slow 40 minutes).
Eőtvős built a revised torsion balance that was a masterpiece of precision engineering. On one end of the hanging rod was a standard platinum mass, while the samples of other materials were suspended from the other end. The rod was mounted on a tripod that could pivot to alter its orientation. A telescope and mirror attached to the moving parts could show if any rotation of the rod had occurred. Tiny imbalances in temperature of the environment could induce warping of the apparatus, leading to spurious rotation, and so the whole assembly was encased in a sealed, insulated chamber. To make the experiments even more exquisitely accurate, the researchers later took to conducting them in a darkened, closed room, so that no light could produce temperature variations. What’s more, they put the device inside a double tent insulated with seaweed.
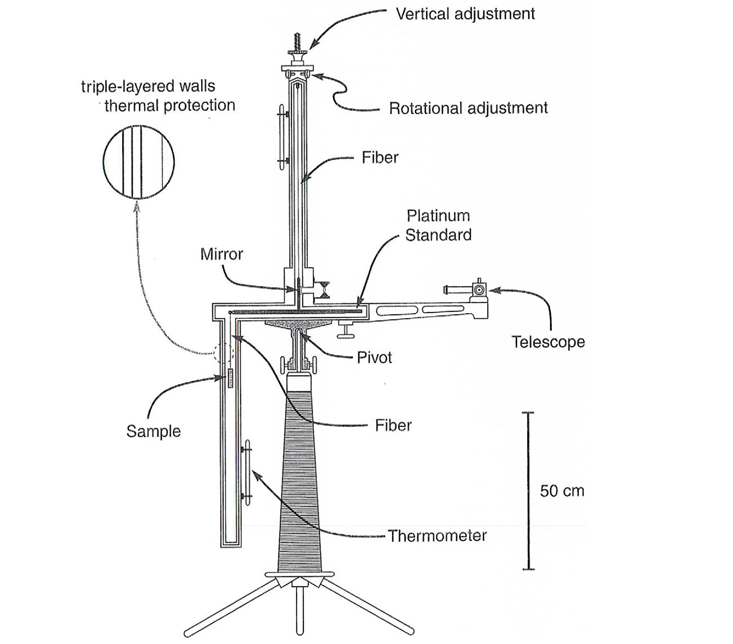
The Hungarian researchers began their torsion balance experiments in 1889, when they found no detectable rotation due to deviations from inertial-gravitational mass equivalence for masses of several different materials, with an accuracy of one part in 20 million.
So by the end of the 19th century, there seemed to be no reason to doubt the weak equivalence principle. But at that very time, new reasons began appearing. For one thing, the discovery of radioactivity suggested the presence of an unknown source of energy locked inside atoms. What’s more, Einstein’s theory of special relativity offered a new perspective on matter and mass. Mass, it seemed, could be converted to energy—and it was sensitive to velocity, increasing as the speed of an object approached the speed of light. Mindful of all this, in 1906 the Royal Scientific Society of Göttingen in Germany offered a 4,500-mark prize for more sensitive tests of the equivalence of “inertia and gravitation,” citing Eőtvős’ experiments as inspiration.
It began to seem as though Fischbach was the discoverer of something non-existent.
Eőtvős himself couldn’t resist returning to the fray. “He was the world expert in this kind of experiment,” says Fischbach. He and his students Dezső Pekár and Jenő Fekete in Budapest dusted off their torsion-balance experiments, devoting thousands of hours to testing different materials: copper, water, asbestos, dense wood, and more. They submitted their findings in 1909, claiming an improved accuracy of one part in 200 million. But the full report of the work wasn’t published until 1922, three years after Eőtvős’ death. Another of his students, János Renner, continued the work and published it in Hungarian in 1935, claiming to verify the weak equivalence principle to one part in 2-5 billion.
Was such sensitivity really possible back then? Physicist Robert Dicke, a specialist in general relativity, expressed doubts when he came to tackle the same question in the 1960s. Regardless of whether Dicke’s criticisms are valid, he and his coworkers used a more sophisticated torsion balance that achieved an accuracy of one in 100 billion. They did it by measuring the acceleration of their test masses caused not by the Earth’s gravity but by that of the sun. This meant there was no need to disturb the balance by rotating it: The direction of the gravitational attraction was itself being rotated as the Earth moved around the sun. Any deviation from weak equivalence should have showed up as a signal varying every 24 hours in step with the Earth’s rotation, giving a precise way to discriminate between this and false signals due to local gravitational variations or other disturbances. Dicke and his colleagues saw no sign of such deviations: No indication that Newton’s law of gravity needed amending with a fifth force.
Were physicists satisfied now? Are they ever?
Fischbach became interested in the fifth force after hearing about an experiment performed by his Purdue colleague Roberto Colella and coworkers in 1975, which looked at the effects of Newtonian gravity on subatomic particles. Fischbach wondered whether it would be possible to conduct similar experiments with subatomic particles in a situation where the gravity is strong enough to make general relativity, rather than Newton’s theory, the proper description of gravity—that might then offer a completely new way of testing Einstein’s theory.
He began to think about doing so using exotic particles called kaons and their antimatter siblings anti-kaons, which are produced in particle accelerators. Analyzing studies of kaons at the Fermilab accelerator facility near Chicago led Fischbach to suspect that some kind of new force might be affecting the particles’ behavior, which was sensitive to a quantity called the baryon number, denoted B.
This is a property of fundamental particles that, unlike mass or energy, doesn’t have any everyday meaning. It is equal to a simple arithmetic sum of the number of even more fundamental constituents called quarks and antiquarks that make up the protons and neutrons of atomic nuclei. Here’s the thing, though: If this new force depended on baryon number, it should depend on the chemical composition of materials, since different chemical elements have different numbers of protons and neutrons. More precisely, it would depend on the ratio of B to the masses of the component atoms. Naively it might seem that this ratio should be constant for everything, since atomic mass comes from the sum of protons and neutrons. But actually a small part of the total mass of all those constituents is converted into the energy that binds them together, which varies from atom to atom. So each element has a unique B/mass ratio.
A force that depends on composition … well, wasn’t that what Eőtvős had been looking for? Fischbach decided to go back and look closely at the Hungarian baron’s results. In the fall of 1985, he and his student Carrick Talmadge calculated the B/mass ratio for the substances in the samples of Eőtvős and his students. What they found astonished them.
The Hungarian team had found very small deviations for the measured gravitational acceleration of different substances, but apparently lacking any pattern, suggesting that these were just random errors. But when Fischbach and Talmadge plotted these deviations against the B/mass ratio, they saw a straight-line relationship, suggesting a force that induced a very small repulsion between masses, weakening their gravitational attraction.
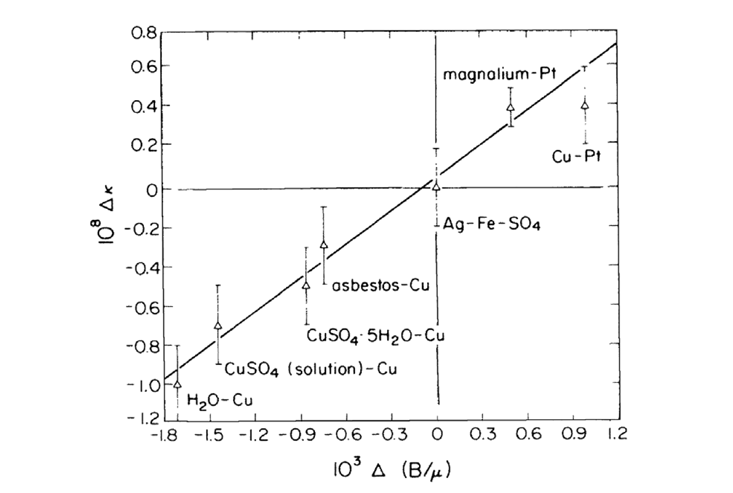
The chemical composition of Eőtvős’ samples wasn’t always easy to deduce—for snakewood and “suet,” who could be sure?—but as far as they could see, the relationship stood up. In one of the most striking cases, platinum and copper sulfate crystals turned out to have the same deviation. Everything about these two substances (density and so forth) are different—except for their near-identical B/mass ratio.
Fischbach and Talmadge presented these findings in their headline-grabbing 1986 paper, helped by postdoc Peter Buck whose command of German enabled him to translate the original 1922 report by Eőtvős’ team. The Purdue group’s paper was reviewed by Dicke, who voiced some doubts but felt eventually that it should be published. Dicke later followed up with a paper claiming that the anomalies in the Eőtvős measurements could be explained by temperature gradients in the apparatus. It was hard, though, to see how such everyday environmental effects would end up producing such a convincing-looking correlation with a quantity as exotic as baryon number.
Once the word was out, the world came calling—not only The New York Times but also the legendary Richard Feynman, whose call to Fischbach’s home four days after the paper was published he initially assumed to be a prank. Feynman was unimpressed, and said as much both to Fischbach and in the Los Angeles Times. But for him to show interest at all showed how the Purdue team’s provocative result had got folks talking.
Considering that our paper was suggesting the presence of a new force in nature,” wrote Fischbach, “it may seem surprising that the referring process went as smoothly as it did.” But maybe the path was smoothed by the fact that there were already both theoretical and experimental reasons to suspect a fifth force might exist.
Back in 1955, the Chinese-American physicists T.D. Lee and C.N. Yang, who shared a Nobel prize two years later for their work on fundamental particle interactions, explored the idea of a new force that depended on baryon number, and had even used Eőtvős’ work to set limits on how strong it could be. Lee met Fischbach just over a week after his paper was published, and congratulated him on it.
What’s more, in the late 1970s two geophysicists in Australia, Frank Stacey and Gary Tuck, had made an accurate measurement in a deep mine of the gravitational constant that relates force to masses in Newton’s equation of gravitational attraction. They reported a value significantly different from that measured previously in laboratories. One way of explaining those results was to invoke a new force that acted over distances of a few kilometers. Stacey and Tuck’s measurements were themselves partly inspired by work in the early 1970s by Japanese physicist Yasunori Fujii on the possibility of “non-Newtonian gravity.”
That’s simply the way physics has always worked: When all else fails, you place a new piece on the board and see how it moves.
After 1986 the hunt was on. If a fifth force indeed acted over distances of tens to thousands of meters, it should be possible to detect deviations from what Newtonian gravity predicts about free fall high above the Earth’s surface. In the late 1980s a team at the United States Air Force laboratory at Hanscom in Bedford, Massachusetts, measured the acceleration due to gravity up a 600-meter television tower in North Carolina and reported evidence for what seemed to be in fact a “sixth force,” for in contrast to Fischbach’s repulsive fifth force it seemed to enhance gravity. After subsequent analysis, however, these claims evaporated.
The most extensive studies were conducted at the University of Washington in Seattle by a team of physicists who, playing on the proper Hungarian pronunciation of “Eőtvős” (close to “Ert-wash”), called themselves the Eot-Wash group. They were co-led by nuclear physicist Eric Adelberger, who “has by now become the world’s leading experimentalist in searching for deviations from the predictions of Newtonian Gravity,” according to Fischbach. The Eot-Wash team used state-of-the-art torsion balances, taking all manner of precautions to eliminate artifacts from their measurements. Result: nothing.
One of the most evocative and suggestive experiments was begun right after the 1986 announcement, by Peter Thieberger of Brookhaven National Laboratory in Upton, New York. He floated a hollow copper sphere in a tank of water and placed it near the edge of a cliff. In 1987 Thieberger reported that the sphere consistently moved in the direction of the edge, where the gravitational attraction by the surrounding rock was smaller—just what you’d expect if there was indeed some repulsive force that counteracted gravity. This was the only corroborating evidence for a fifth force published in a prominent physics journal. Why did it alone see such a thing? That’s still a mystery. “It is not clear what—if anything—was wrong with Thieberger’s experiment,” wrote Fischbach.
By 1988 Fischbach counted no fewer than 45 experiments searching for a fifth force. Yet five years later only Thieberger’s had produced any sign of it. In a talk to mark the tenth anniversary of the 1986 paper, Fischbach admitted that “There is at present no compelling experimental evidence for any deviation from the predictions of Newtonian gravity … the preponderance of the existing experimental data is incompatible with the presence of any new intermediate-range or long-range forces.”
It began to seem as though, as Fischbach ruefully puts it, he was the discoverer of something non-existent. The mood was captured by physicist Lawrence Krauss, then at Yale University, who responded to the 1986 paper by formally submitting to Physical Review Letters a spoof paper claiming to have re-analyzed Galileo’s experiments on the acceleration of balls rolling downhill under gravity, reported in his 1638 book Discourses on Two New Sciences, and to have found evidence for a “third force” (in addition to gravity and electromagnetism). The paper was rejected by the journal in the same spirit as it was submitted: on the basis of six spoof referees’ reports clearly written in house.
After a few decades of almost universal non-detection of a fifth force, you might think the game is over. But if anything, reasons to believe in a fifth force have become ever more attractive and diverse as physicists seek to extend the foundations of their science. “There are now thousands of papers suggesting new fundamental interactions that could be a source of a fifth force,” says Fischbach. “The theoretical motivation is quite overwhelming.”
For example, the latest theories that attempt to extend physics beyond the “standard model,” which accounts for all the known particles and their interactions, throw up several possibilities for new interactions as they attempt to uncover the next layer of reality. Some of those theories predict new particles that could act as the “carriers” of previously unknown forces, just as the electromagnetic, strong, and weak forces are known to be associated with “force particles” such as the photon.
A group of models predicting deviations from Newtonian gravity called Modified Newtonian Dynamics (MOND) have also been put forward to account for some aspects of the movements of stars in galaxies that are otherwise conventionally explained by invoking a hypothetical “dark matter” that interacts with ordinary matter only (or perhaps almost only) via gravitational attraction. No clear evidence has been discovered to support MOND theories, but some physicists have found them increasingly promising as extensive searches for dark-matter particles have yielded no sign.
Were physicists satisfied now? Are they ever?
Alternatively, says Feng, a fifth force might help us find out about dark matter itself. As far as we know, dark matter only interacts with other matter through gravity. But if it turned out to feel a fifth force too, then, Feng says, “it could provide a ‘portal’ through which we can finally interact with dark matter in a way that is not purely gravitational, so we can understand what dark matter is.”
What’s more, some theories that invoke extra dimensions of space beyond our familiar three—such as the currently most favored versions of string theory—predict that there could be forces similar to but considerably stronger than gravity acting over short distances of millimeters or less.
That’s the scale at which some researchers are now looking. It means measuring the forces, with extraordinary precision, between small masses separated by very small gaps. Three years ago Fischbach and colleagues set out to do this for tiny particles just 40 to 8,000 millionths of a millimeter apart. The difficulty with such measurements is that there is already a force of attraction between objects this close, called the Casimir force. This has the same origin as the so-called van der Waals forces that operate at even closer approach, and which stick molecules together weakly. These forces come from the synchronized sloshing of clouds of electrons in the objects, which give rise to electrostatic attraction because of the electrons’ charge. Casimir forces are basically what van der Waals forces become when the objects are far enough apart—more than a few nanometers—for the time delay between the electron fluctuations across the gap to matter.
Fischbach and his coworkers found a way to suppress the Casimir force, making it about a million times weaker by coating their test masses with a layer of gold. They attached a gold-coated sapphire bead about 150 thousandths of a millimeter in radius to a solid plate, whose motions could be detected electronically. Then they rotated a microscopic disk patterned with patches of gold and silicon just below the bead. If there were any differences in the force exerted by the gold and silicon, that should produce a vibration of the bead. They saw no such effects, which meant they could place even more stringent limits on the possible strength of a material-dependent fifth force at these microscopic scales.
Torsion-balance measurements can be used in this region, too. Researchers at the Institute for Cosmic Ray Research at the University of Tokyo have used the device to look for deviations from the standard Casimir force caused by a fifth force. All they found were yet stricter lower limits on how strong such a force can be.
As well as detecting a fifth force directly, it might still be possible to spot it the way Fischbach originally thought to look: through the high-energy collisions of fundamental particles. In 2015 a team at the Institute for Nuclear Research in Debrecen, Hungary, led by Attila Krasznahorkay, reported something unexpected when an unstable form of beryllium atoms, formed by firing protons at a lithium foil, decays by emitting pairs of electrons and their antimatter counterparts positrons. There was a rise in the number of electron-positron pairs ejected from the sample at an angle of about 140 degrees, which standard theories of nuclear physics couldn’t explain.
The results were all but ignored until Feng and his coworkers suggested last year that they could be accounted for by the ephemeral formation of a new “force particle” which then quickly decays into an electron and a positron. In other words, this hypothetical particle would carry a fifth force, with a very short range of just a few trillionths of a millimeter.
Although they haven’t yet been replicated by other researchers, the Hungarian findings look pretty solid. The chance that they are just a random statistical fluctuation is tiny, says Feng: about 1 in 100 billion. “More than that, the data fit beautifully the hypothesis that they’re caused by a new particle,” he says. “If such a new particle exists, this is exactly how it would come to light.” Schlamminger agrees that Feng’s interpretation of the Hungarian observations was “one of the exciting things that happened in 2016.”
“We have yet to confirm it is a new particle,” admits Feng, “but it would be revolutionary if true—the biggest discovery in particle physics in at least 40 years.” His theoretical work predicts that the putative new particle is just 33 times heavier than the electron. If so, it shouldn’t be hard to make in particle collisions—but it would be hard to see. “It is very weakly interacting, and we’ve shown that it would have eluded all previous experiments,” says Feng. Perhaps, he adds, it could be sought at colliders such as the Large Hadron Collider at the particle-physics center CERN in Geneva.
The hypothesis of a fifth force is, then, anything but exhausted. In fact it’s fair to say that any observations in fundamental physics or cosmology that can’t be explained by our current theories—by the Standard Model of particle physics or by general relativity—are apt to get physicists talking about new forces or new types of matter, such as dark matter and dark energy. That’s simply the way physics has always worked: When all else fails, you place a new piece on the board and see how it moves. Sure, we haven’t yet seen any convincing evidence for a fifth force, but neither have we seen a direct sign of dark matter or supersymmetry or extra dimensions, and not for want of looking. We have ruled out a great deal of the territory that a fifth force might inhabit, but there is still plenty of terrain left in shadow.
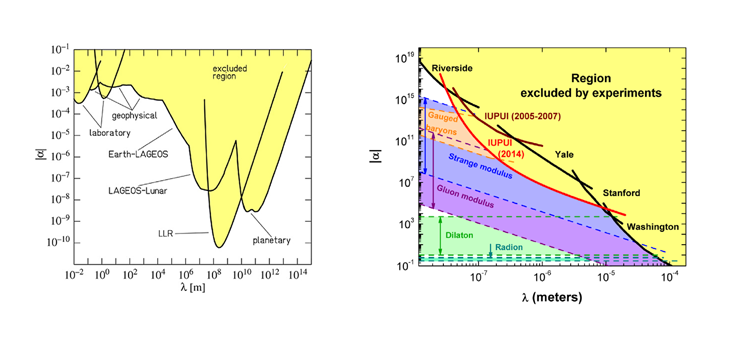
At any rate, the search continues. In April 2016, the European Space Agency launched a French satellite called Microscope that aims to test the weak equivalence principle in space with unprecedented accuracy. It will place two nested pairs of metal cylinders in free fall: One pair is made of the same heavy platinum-rhodium alloy, the other has an outer cylinder of lighter titanium-vanadium-aluminum. If the cylinders fall at a rate that depends ever so slightly on the material—so that deviations from the weak equivalence principle occur at a level of one part in a thousand trillion, about 100 times smaller than is detectable in current Earth-based experiments—it should be possible to measure the differences with electrical sensors on the satellite.
“String-theory models predict WEP violations below one part in 10 trillion,” says Joel Bergé, a scientist at the French Centre for Aerospace Research (ONERA) that manages the Microscope project. He says that the scientific operations of the mission began last November and the first results should be published this summer.
Despite such high-tech studies, it’s the Eőtvős torsion-balance experiments that Fischbach keeps returning to. Back then, the Hungarians had no theoretical motivation to expect a composition-dependent fifth force—nothing that could have subconsciously swayed them in their incredibly delicate work. “Whatever we need to explain their data simply didn’t and couldn’t conceptually exist then,” says Fischbach. And yet they did seem to see something—not a random scatter of results, but a systematic deviation. “I keep thinking, maybe I’m missing something about what they did,” says Fischbach. “It’s still a puzzle.”
Philip Ball is a writer based in London. His latest book is The Water Kingdom: A Secret History of China.