Space should be churned up like a speedboat-filled lake, crisscrossed by gravitational waves rushing at the speed of light in every direction. That’s because any kind of acceleration, of any kind of mass, will produce a gravitational wave. When you whoosh your arm through the air, you are launching a gravitational wave that will travel forever. The Earth produces gravitational waves as it orbits the sun. So do black holes that twirl around or crash into each other.
Every accelerating mass produces a signal, and all those signals should add together into a detectable background.
So where is it? Scientists have been trying to tune in to the staticky drone of gravitational wave background noise for years. An experiment that uses the timing of distant pulsars has been running for over a decade, searching for the portion of the background due to pairs of supermassive black holes. But they haven’t heard a peep.
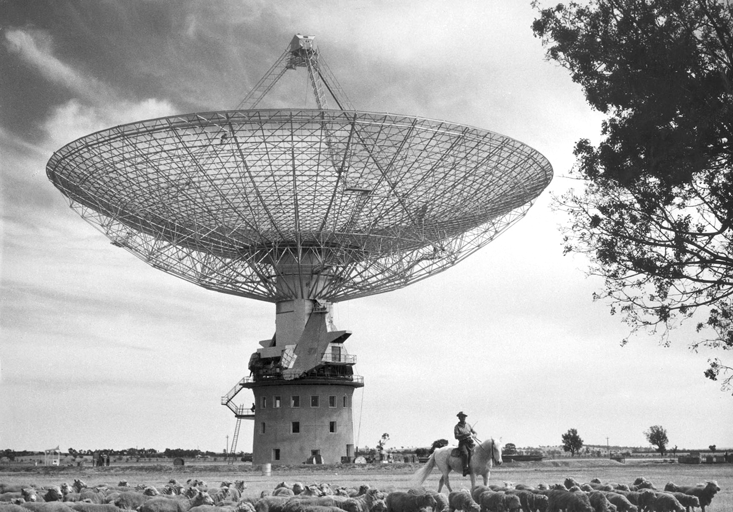
Then, early this year, the Laser Interferometer Gravitational-Wave Observatory (LIGO) achieved a positive detection of a single gravitational wave event, resulting from the merger of lighter, stellar-mass black holes. The more subtle mission of the pulsar timing experiments and their search for background seemed to get drowned out. They have, after all, produced a null result.
But sometimes silence speaks volumes.
Gravitational waves come in different frequencies, just like light waves. Their frequency is based on their motion—objects in a year-long orbit, no matter their mass, will make waves with the same frequency (though lighter objects will produce a lower-amplitude wave).
Some gravitational-wave sources are strong and close enough that scientists can pick up individual events, like the 200-hertz-frequency “chirp” they detected at LIGO this February, which happened when two black holes around 30 times the mass of the sun merged into one. Others are distant and hard to resolve individually—like close-orbiting, destined-to-merge pairs of supermassive black holes, which can be billions of times bigger than the sun and are often billions of light-years away. These latter, in aggregate, should create a constant background at a much lower frequency than what LIGO can pick up.
“Imagine a gravitational-wave background as being like the surface of the ocean, and we’re on the Earth on a boat.”
It was in the summer of 1967 that astronomer Jocelyn Bell first saw the signal that would give scientists the tools they needed to listen in on this background. She had been hunting for distant galaxies with a radio telescope, when a spike rose above the baseline noise in her data, a pulse of radio waves that reappeared every 1.3 seconds. It looked like a steady heartbeat on an EKG. She was mystified by the regular blip-blip-blip of it. The only objects she knew that could produce such fast, reliable signals were synthetic. She and her advisor, Anthony Hewish, half-jokingly suggested they were looking at a message from aliens, and dubbed the source of the radio waves LGM-1 for “Little Green Man 1.”
Soon, though, astronomers discovered that the signal was coming from something almost as bizarre as aliens—a neutron star, a city-sized star made mostly of crushed-close neutrons that is the remnant left behind after a supernova. Around the time that Bell found her strange signal, two astronomers—Franco Pacini and Thomas Gold—noted that a spinning neutron star surrounded by a magnetic field could emit radiation (although, to this day, scientists cannot explain all the details of why). Gold connected this to Bell’s discovery, explaining how the spin could periodically point a beam of radiation at Earth, making a pulse blip across our telescopes.
Neutron stars can spin around hundreds of times per second, sweeping their beams across space as they do. If these beams happen to be aligned with the Earth, they would briefly illuminate our planet like a distant lighthouse. When scientists found a second pulsing source in 1968, the connection was confirmed: It was located in the middle of the Crab Nebula, which is gas left over from a supernova explosion.
Pulsar clocks are extremely reliable. Because they are so dense, so spherical, and have so much spin momentum, almost nothing can change their rotation rate. The timing of their lighthouse sweep is remarkably constant, earning them the moniker “nature’s best clocks.” The most precise ones—which are also the fastest, called millisecond pulsars—slow their spins by just a few picoseconds per year. By comparison, the most precise atomic clock ever created loses about 66 picoseconds a year.
By 1979, astronomers had realized that they—or, really, someone else in the future with better telescopes—could use these strange, ultra-precise clocks to detect gravitational waves. Steven Detweiler of the University of Florida in Gainesville and Mikhail Vasilievich Sazhin of Moscow State University independently discovered that if a gravitational wave passed over a pulsar, or the Earth, the time at which the pulsar’s emissions arrived at the Earth would change. Astronomers wouldn’t get the tick-tick-tick at the hyper-regular intervals they expected.
“If a gravitational wave passes through the pulsar, it changes the effective distance to that pulsar, rocking it back and forth,” says Maura McLaughlin of West Virginia University in Morgantown, and the former chair of the North American Nanohertz Observatory for Gravitational Waves (NANOGrav). That changes how far the emissions have to travel and when they arrive at our planet. The same thing happens if a wave passes through the Earth.
For a tens of billions of solar masses pair of supermassive black holes hundreds of millions of light-years away, a millisecond pulsar’s pulse arrival time would change by microseconds. But most such binaries are expected to be farther away and less massive, and the skew would be just tens of nanoseconds or smaller. In Detweiler and Sahzin’s time, telescope instruments could not take and dump data that fast; computers could not store and process the terabyte-level output; and no one had yet discovered any millisecond pulsars. They needed something else.
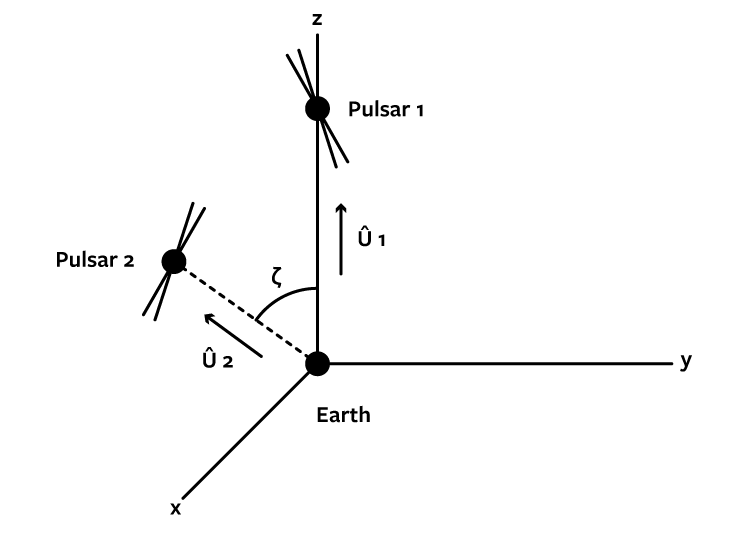
In 1982, Ronald Hellings of the Jet Propulsion Laboratory and George Downs of the California Institute of Technology, both in Pasadena, made a breakthrough: They suggested scientists look at lots of pulsars at once, and use their collective late-and-early arrivals to detect the whole noisy gravitational-wave background at once—not individual disturbances. They modeled how that staticky background, buzzing across the universe, would show up in the blips of a bunch of pulsars, which scientists later called a “pulsar timing array.”
Try picturing it, suggests Chiara Mingarelli of the California Institute of Technology in Pasadena. “You can imagine a gravitational-wave background as being like the surface of the ocean,” she says. “And we’re on the Earth on a boat, and we’re bobbing up and down in this gravitational-wave sea.”
So are the pulsars, but their bobbing in the sea looks like pure noise, says McLaughlin, “because they are all happening at different times and are hence uncorrelated.”
It would describe not just individual objects—like the LIGO detection did—but also the formations and evolutions of entire galaxy populations.
But the bobbing from Earth, while it’s noisy, has some structure. That structure is what Hellings and Downs mapped out. When gravitational waves make the Earth bob, they change the arrivals of flashes from all pulsars at the same time. Gravitational waves squeeze in one direction, compressing spacetime, while stretching in the other, expanding it. Imagine that along a north-south line, space condenses. At the same time, east-west expands. Two pulsars in the northern direction of our sky would show similar speedups in the timing of their blips, and two pulsars in an eastern direction would have similar slowdowns.
Hellings and Downs laid out how these late and early arrivals should match up with pulsars spread across the sky. Using their predicted signature and a passel of pulsars, the scientists were able to gain sensitivity over looking at single pulsars. The Hellings-Downs Curve, as the signature signal is now called, is still what astronomers look for today. At the time that Hellings and Downs did their work, though, the technology wasn’t good enough, and astronomers had not discovered any ultra-precise millisecond pulsars. “There was no way,” says McLaughlin. They would need to bury their technique into a time capsule for the future to find.
But they also realized the potential for new science. No one was close to a direct detection of gravitational waves, and LIGO wouldn’t receive its first funding for 12 more years. Pulsar astronomers had a shot at being the first to prove beyond doubt that gravitational waves exist. And on top of that, they could use those gravitational waves to learn about how the universe came to be the way it is. They knew how to be sensitive to the signal, and they knew computers would catch up to the processing speeds the project needed.
Throughout the ’80s and ’90s, people continued work on the gravitational-wave background—in the background. “But they hadn’t really been giving it all they had because we weren’t at the level where we could really expect to make a detection,” says McLaughlin.
Challenging scientists to reconsider their notions of galaxy formation could lead to interesting new science.
But as the years passed, telescope instruments gained more processing power. New pulsars piled up. While astronomers knew of just four millisecond pulsars in the 1980s, they found 31 more in the 1990s, and 65 more between 2000 and 2010. They have discovered 150 since then, bringing the total to 250.
In 2005, Dick Manchester of Australia Telescope National Facility decided it was time to act. He and his colleagues founded the first pulsar search for background gravitational waves: the Parkes Pulsar Timing Array. Using the pastorally located 209-foot Parkes Telescope in New South Wales, which often has sheep meandering beneath its dish, the team began its search. They collected blip after blip from 20 of the most precise pulsars, watching them like airport departure/arrival screens, searching for the Hellings-Downs Curve.
Farther north, astronomers formed the European Pulsar Timing Array later the same year, catching pulsar radio waves with five different telescopes in Effelsberg, Germany; Cheshire, England; Nançay, France; Pranu Sanguni, Italy; and Westerbork, the Netherlands. Each measures 210 to 330 feet across, and they are still running today, keeping time with 18 high-precision pulsars. Because only 24 hours exist in a day, and most telescopes aren’t all-pulsars-all-the-time, having more telescopes involved allows astronomers to spread more observations across instruments.
In the United States, pulsar astronomers were behind, but they had a secret weapon. For telescopes, bigger is better. And like American sodas, American telescopes outweighed the competition. U.S. astronomers had access to the Arecibo Telescope in Puerto Rico, which measures 1,000 feet across, and the Green Bank Telescope in West Virginia, which is 328 feet wide, compared to Europe’s 330-footers and Australia’s single 209-foot dish.
Fred Lo, the director of the National Radio Astronomy Observatory, which operates the Green Bank Telescope, wanted to take advantage of that size difference. In 2008, he called together a group of prominent pulsar scientists who worked at or used his observatory, like McLaughlin, Duncan Lorimer of West Virginia University, and observatory scientist Scott Ransom. Each scientist was working on his or her individual projects, chipping away on their own favorite pulsars. He connected them, telling them to get their act together and start collaborating, and join the hunt for the gravitational-wave background.
“At that time we picked an acronym,” McLaughlin continues. “The most important part, of course.” They called themselves NANOGrav, for the North American Nanohertz Observatory for Gravitational Waves.
Each of the three teams collected and analyzed data from the telescopes they were most familiar with, by virtue of their geographical proximity and federal funding sources. But they all knew that if they combined their work, they would have a better shot at sensing the waves sooner. All three groups linked up in 2009 to form a network of networks: the International Pulsar Timing Array (IPTA). Using a list of 39 of the best pulsars, they got to work. Today, that list has grown to roughly 100. And while some competition exists among pulsar-precisionist groups, the scientific benefits of sharing data outweigh the costs. “There are people who really want to be the ones to do it and to get the glory, but I think that is a small group of people,” says McLaughlin. “Nearly everyone has accepted that the first detection will come from IPTA data.”
In a sense, though, NANOGrav has already produced new science, even without recording a single bit of signal.
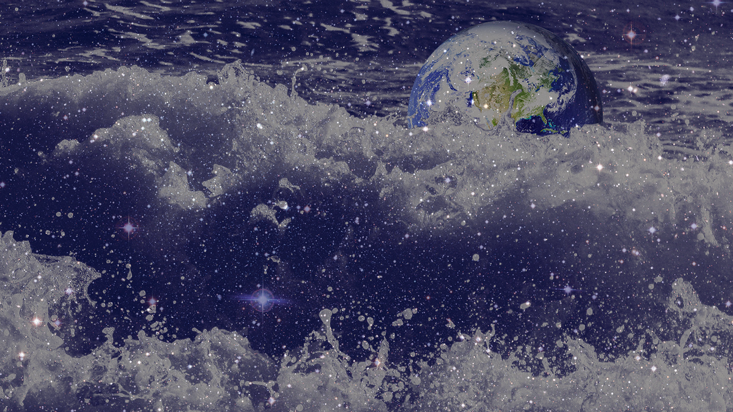
What’s not widely appreciated is that the silence from pulsar timing array experiments is some of the first science—beyond “we found them!” or “we didn’t find them!”—to come out of decades of experimental gravitational-wave work. That’s why McLaughlin gets upset when the LIGO discovery looms so large that no other research seems important. “I’ve had several people say, ‘So are you guys just going to give up now?’ ” she says. “I’m like, ‘Noooo, that’s not the point.’ ”
Because the gravitational background noise that NANOGrav is searching for would come from a whole population of supermassive black holes, it would describe not just individual objects—like the LIGO detection did—but also the formations and evolutions of entire galaxy populations. As a result, the size of the signal reflects some of the basic features of our universe.
To estimate the size of that signal, scientists used models of how many double supermassive black holes the universe holds, how big they are, how fast they whip around each other, and where they are. These estimates reflected the state of the art understanding about how galaxies form, how they change over time, and how they get bigger. The conclusion was that, if they monitored around 20 pulsars for five to 10 years, their sensitivity should be sufficient to hear the nanohertz gravitational background drone. When, 11 years after array initiation, they still had found nothing, they effectively learned that some of those initial assumptions were wrong. All three teams estimated in 2015 that the actual noise amplitude had to be at least 10 times lower than their initial estimates.
This lowering of expected signal strength was a kind of anti-news, the opposite of LIGO’s historic detection. But challenging scientists to reconsider their notions of galaxy formation and evolution could lead to interesting new science. Perhaps fewer galaxies host big black holes in their centers than scientists thought—and right now scientists think that almost all substantial (non-dwarf) galaxies do. Maybe galaxy mergers are less frequent than was estimated. (Right now, says Mingarelli, they are trying to figure out what “fewer” actually means.) Or maybe the time between the first encounter between two black holes and their coalescence doesn’t quite follow the equation theorists have developed. It could also be that most black hole mergers stall out somehow before the holes are close enough to emit swelling (detectable) gravitational waves, and that the pair just keep orbiting each other endlessly and never merge. Or maybe scientists have been sizing supermassive black holes all wrong, and they are smaller than once thought, so that their waves are smaller. Right now, all of these scenarios are in play as possibilities.
Of course, the goal remains to make an actual detection. Based on new calculations from their nine-year dataset, NANOGrav estimates that they will reach the sensitivity necessary to finally hear the static in another five to 10 years. In their latest paper, their sensitivity estimates include adding four new hyperstable pulsars each year, taking them from 54 to around 100. “I think pulsar-timing is ready in terms of people, techniques, and analysis,” says Michele Vallisneri, a member of the LIGO collaboration, a visiting associate at the California Institute of Technology, and a research scientist at the Jet Propulsion Laboratory. But, he cautions, it is also possible that “nature may have put our goal farther than we think it is.”
Time to detection also depends on something more down-to-Earth: funding. “[If] we lose access to either the Green Bank Telescope or Arecibo, the time to detection is pushed back several years … and possibly forever if we lose both,” says McLaughlin. The National Science Foundation will stop funding Green Bank in 2017 or 2018, and the observatory is pursuing private partnerships. At Arecibo, threats of closure have popped up for years—including this summer, as one also did for Parkes—but both telescopes remain open. Those in Europe are, so far, safe.
Whatever happens, NANOGrav is one example of what will become many categories of instruments to complement LIGO’s initial discovery, says Vallisneri. As he puts it, “astronomers didn’t stop looking after Galileo first saw the satellites of Jupiter and the phases of Venus.”
Sarah Scoles is a writer based in Denver, Colorado, and a contributor at Wired Science.
The lead photocollage was created with images from: ESO/G. Bono & CTIO and Pixabay