Neutrino physics is full of unusual characters. There was Ettore Majorana, who disappeared in 1938 without a trace, taking his savings with him. No record of him has ever been found, though there have been numerous disputed sightings of him throughout the years.
Then there was Bruno Pontecorvo. Suspected of slipping nuclear secrets out of England, he vanished while on vacation in Italy in 1950 and reappeared five years later singing the praises of his new homeland: the Soviet Union.
Strangest of all, though, is the neutrino itself. It is electrically neutral, making it invisible to particle detectors, and bizarrely lightweight, at most 0.0004 percent the weight of the next-lightest particle, the electron. Although it is the most numerous massive particle in the universe, it is so slippery that it can pass through a light year of lead as if it wasn’t there. And then there is the matter of the shape-shifting.
Neutrinos come in three flavors: electron, muon, and tau, each named for the charged particle with which it is associated. But the flavors are not pure essences—each is made up of a different combination (or superposition) of three ingredients, or mass states.
How they get mass is a physicist’s version of the Zen koan pondering the sound of one hand clapping.
These mass states behave not as simple dumbbells of differing weights, but as waves of differing lengths. Because the waves do not line up with each other perfectly, at different points the height of one mass state will vary with respect to that of the other two. That means that sometimes the combination of mass states will most resemble the recipe for an electron neutrino, while at other times it will look like that of a muon neutrino. As a result, neutrinos appear to oscillate among the three flavors as they travel.
No other fundamental particles do this. “Only the neutrinos can change from one type to another,” says André de Gouvêa of Northwestern University in Evanston, Illinois. More than a quirk of nature, this ability to mutate on the fly points to some deep questions in physics, and potentially, some important answers.
Neutrino mutation would not be possible if it weren’t for the particle’s minuscule mass. Because each of the three known mass states is so small and its associated quantum wavelength is so long, the waves corresponding to each state can remain largely in sync, with only small offsets, over cosmic distances. This allows neutrinos to flicker between different flavors in an ephemeral state of multiplicity.
If their masses were larger and their wavelengths shorter, the waves would quickly become so out of phase that this knife-edge balance between different flavors would collapse, forcing the neutrinos into one type or the other. “The different flavors would separate from each other,” says de Gouvêa. “They would have a very binary behavior.” The fact that neutrinos don’t, thanks to their puny mass states, makes sense according to the rules of quantum mechanics, but it is still mind-bending, says neutrino researcher Jason Koskinen of the University of Copenhagen. “I still haven’t wrapped my head around this,” he admits.
There is just one snag: Neutrinos weren’t supposed to have any mass at all. “We built our standard model around the idea that neutrinos are massless,” says Janet Conrad of the Massachusetts Institue of Technology (MIT).
The fact that they have mass, however small, is a big problem. The standard model is physicists’ best idea of how particles and forces interact—a spectacularly strong edifice whose construction was completed in 2012 with the discovery of its last missing particle, the Higgs boson. “Neutrino oscillation is the only confirmed physics right now that can be done outside the standard model,” says Koskinen.
The reason that neutrino mass is so tricky has to do with how any particle gets its mass. Other elementary particles with mass come in two mirror versions—one left- and one right-handed—that correspond to the direction of their spin. Each version can interact with a different force of nature, and both “hands” seem to be required to give particles mass, thanks to their interaction with an invisible quantum “ether” that suffuses all of space: the Higgs field, whose signature particle is the Higgs boson.
The Higgs field acts a bit like a mirror, turning a particle with one spin into its mirror opposite. “The idea is that every once in a while, a left-handed particle will hit the Higgs field and convert to a right-handed particle,” says de Gouvêa. “The net effect is that it looks like a particle with mass.”
It is so rare that it is typically expected to occur on timescales much longer than the age of the universe.
Neutrinos, by contrast, interact only with the one-handed weak nuclear force (and technically gravity, but the strength of this force compared to the others is negligible). And indeed, only left-handed neutrinos have been observed. If neutrinos have no mirror reflection, they should have no mass, according to the standard model, so how they get mass is a physicist’s version of the Zen koan pondering the sound of one hand clapping. “Many particle physicists who work on the subject get confused about it,” says de Gouvêa.
One possibility is that neutrinos do have a reflection, but one that only they can see. That is, there are right-handed neutrinos, but their presence has not been detected because they are even more aloof than their southpaw counterparts and have no mass. “That particle doesn’t participate in any force,” says de Gouvêa of the purported right-handed neutrino. “It literally does not interact with anything, except with the left-handed neutrino to give it a mass.”
How neutrinos gain their mass is a mystery whose solution promises to spill over the boundaries of neutrino physics itself, and into one of the biggest questions of cosmology: Why is there more matter in the universe than antimatter? According to the standard model, equal amounts of matter and antimatter should have been made after the big bang. When matter and antimatter meet, they instantly and completely annihilate each other. So the big bang should have led in quick succession to a great conflagration. The fact that we are here today shows that some process tipped the scales to leave behind more matter. “How did equality evolve into inequality?” asks Boris Kayser, a neutrino theorist at Fermilab in Batavia, Illinois. “Matter and antimatter have to behave differently.” Many physicists suspect neutrinos played a role in this imbalance—but if they do, it’s unlikely that they get their mass the way other particles do (interacting with the standard Higgs field through a right-handed version of themselves).
Fortunately, there’s a loophole, raised nearly 80 years ago by the enigmatic Majorana. Instead of invoking a separate right-handed matter neutrino, the neutrino anti-particle (the antineutrino) could act as a mass partner for its lefty counterpart. After all, the antineutrino is right-handed. For this to work, though, neutrinos would need to be their own antiparticles. That means that if two neutrinos ever met each other, they would instantly annihilate.
One way to test whether this is happening is to look for radioactive particle decays that should leave behind signs of two antineutrinos but don’t—presumably because the antineutrinos, being their own antiparticles, had annihilated immediately after forming. With the exception of one controversial result reported about a decade ago, this signature, known as neutrinoless double beta decay, has yet to be seen. That doesn’t mean the process (two neutrons decaying to produce two protons and two electrons) doesn’t exist: It is so rare that it is typically expected to occur on timescales much longer than the age of the universe.
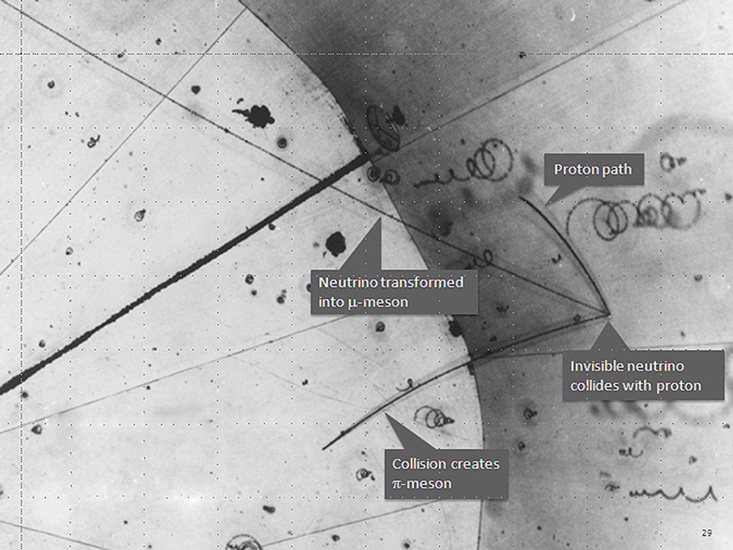
But not always. Statistically, the decay could occur on timescales detectable in the lab. “If somehow we were told we can only look for neutrino masses in one way and only one way, then neutrinoless double beta decay would probably be the highest priority,” de Gouvêa says. Several new hunts, including the Italian CUORE and the Canadian SNO+ experiments, aim to scrutinize the radioactive decays of elements such as tellurium for the telltale absence of antineutrinos.
If neutrinos are not their own antiparticles, neutrinoless beta decay would never happen. Instead, the two neutrons would leave behind two protons, two electrons and two antineutrinos. In that case, the difference between the number of matter and antimatter leptons—that is, neutrinos, electrons, muons, and tau particles—would be zero both before and after the decay. If neutrinos are their own antiparticles, however, two leptons (the electrons) would be left standing after the decay—and no antileptons. The net result would be an increase in the quantity of matter leptons, at the expense of their antimatter counterparts. Similar processes operating in the early universe might provide just the ticket to explain the universe’s disparity between matter and antimatter.
That is a promising direction for cosmologists interested in the nature of the universe. But, it also means some new physics is needed to explain how neutrinos get their mass; the usual interaction among left-handed, right-handed, and Higgs particles would not work.
One idea is that neutrinos have their own Higgs field, a mirror that reflects only neutrinos and no other particles. “It’s like the neutrinos require their own Higgs boson,” says de Gouvêa.
Chian-Shu Chen of the National Center for Theoretical Sciences in Hsinchu, Taiwan, and Ya-Juan Zheng of National Taiwan University in Taipei calculate that it is possible that signs of this new Higgs boson could appear at the Large Hadron Collider in Switzerland. “We expect [the] neutrino mass mechanism could have the chance to be revealed within the reach of the LHC,” says Chen.
But he acknowledges that it would be “very lucky” if that happened, since physicists would ordinarily expect the new particles to be produced at much higher energies than the LHC can reach. Alexei Smirnov of the Max-Planck Institute for Nuclear Physics in Heidelberg, Germany, agrees. “I would call this activity ‘searching under the lamp,’ ” he says. “There is no other serious motivation for this construction but making something observable at the LHC.”
Pontecorvo’s defection contributed to his wife’s nervous breakdown.
Another possibility is to add one or more extra types of neutrino that would be even less sociable than an ordinary one. This is similar to the idea of simply adding a right-handed neutrino, except in this case, the extra neutrino interacts with itself to provide its own mass. It is referred to as a massive “sterile” neutrino, since it can only affect other particles gravitationally. “The left-handed guys have their own mass, and the right-handed guys have their own mass,” says Rabindra Mohapatra of the University of Maryland, College Park.
If a “sterile” neutrino exists, it should have a mass that is inversely proportional to that of the ordinary neutrino, as if the two neutrino types were on opposite sides of a seesaw. And that could help explain a puzzling gap in the distribution of the masses of fundamental particles, says Mohapatra. The quarks that make up protons and neutrons are about 10 times as massive as the electron, but the electron is at least 250,000 times as massive as the next lightest particle, the neutrino. “We were always worried about the fact that the neutrino mass seems to be much smaller than the electron mass,” says Mohapatra. In the seesaw mechanism, which Mohapatra helped originate 35 years ago, the extremely low mass of ordinary neutrinos can be explained if there are very heavy steriles as well.
The seesaw mechanism could possibly produce exotic charged particles that would appear in the detritus of proton collisions at the LHC. Finding evidence of massive sterile neutrinos would be exciting “because it would tell us that neutrino masses are evidence for some other independent source of mass” for fundamental particles besides the ordinary Higgs field, says de Gouvêa. Such a discovery would get at the heart of the origin of mass, one of the most basic questions in physics.
As for Pontecorvo, the man who first suggested that neutrinos might shape-shift, his own life was nothing if not a seesaw. He soon came to regret having defected to the Soviet Union. “After a few years, I understood what an idiot I was,” Pontecorvo told a reporter in 1992, a year before his death. But it was too late. His defection prevented him from traveling abroad for many years, contributed to his wife’s nervous breakdown, and, ironically, shut him out from the nuclear reactor research he had probably left the United Kingdom to pursue, says Pontecorvo biographer Simone Turchetti, a science historian at the University of Manchester. “This really is a story of a man living two completely different lives in two completely different worlds,” Turchetti says—rather like the particle he studied.
Maggie McKee is a freelance science writer focusing mainly on astronomy and physics. Previously an editor at New Scientist and Astronomy magazines, she lives near Boston with her husband.