A team of astronomers announced this year that they had found no fewer than eight planets orbiting the so-called Goldilocks zone around their parent stars, inside which the temperatures are neither too hot nor too cold to support life as we know it on Earth. Two of these, called Kepler-438b and Kepler-442b, are the most Earth-like candidates yet seen among the 1,900 or so exoplanets (planets outside our solar system) now known to exist.
“Earth-like” means, in part, that a planet has a good chance of carrying liquid water—something more likely to be true inside the Goldilocks zone. “Follow the water” has become a mantra for astrobiologists seeking signs of life elsewhere in the cosmos. With the new ability to detect the characteristic fingerprint of water in the light reflected from the atmospheres of exoplanets, some astronomers hope to spot a world that hosts life soon.
But is liquid water really necessary to life?
There’s a long history to the belief that it is. In 1913, the Harvard biochemist Lawrence Henderson proposed a curious inversion of Darwinian evolution, in which organisms become “fit” for their environment by adaptation. Henderson’s book The Fitness of the Environment argued that the cosmic environment is itself peculiarly “fit” to host life.
This was deeply puzzling. How could an environment acquire fitness?
Henderson pointed out that water, in particular, seemed replete with “biocentric” attributes, as if it were uniquely designed as life’s solvent. The fact that it is a liquid at all on Earth is more unusual than it might seem. Other simple hydride molecules—methane, hydrogen sulfide, ammonia, hydrogen chloride— are all gases at room temperature and pressure—but not “oxygen hydride” H2O. There seems to be some extra stickiness binding water molecules together.
And because water has a high heat capacity (it can absorb a lot of heat without much increase in temperature), ocean currents can redistribute huge amounts of solar heat and help make planetary conditions more uniform and stable. What’s more, while most liquids shrink and get denser when they freeze, ice expands and floats. As a result, ponds don’t freeze solid from the bottom up and then become almost impossible to thaw; instead, a frozen lid insulates the water beneath.
Water also dissolves a wide range of substances, so that it helps to carry essential nutrients and elements to organisms that need them. Without water’s exception al ability to host ions (electrically charged atoms and molecules), we wouldn’t have photosynthesis or nerve impulses. The large surface tension of water makes it possible for sap to rise up great distances through capillary action, so that plants can stand tall. And so on.
This was deeply puzzling. How could an environment acquire fitness? After all, the chemical ingredients of a planet—water, rocks, air—don’t mutate and reproduce, the key attribute for achieving Darwinian fitness. Yet some of these vital attributes of water had been noted before. In the mid-19th century, several British scholars were commissioned by the Earl of Bridgewater to write a series of books showing “the Power, Wisdom, and Goodness of God, as manifested in the Creation”—in other words, how God’s wisdom was revealed in the discoveries of science, an objective called natural theology. In one of these Bridgewater Treatises, published in 1834, the English chemist William Prout asserted that liquid water’s expansion close to its freezing point was an example of this divine providence.
Henderson was not so ready to cede ground to God, but he admitted that it was not easy to find any alternative explanation for water’s apparent “fitness.” All he could say was that there was “exceedingly little ground for hope that any single explanation of these coincidences can arise from current hypotheses and laws.” If they are ever to be understood, he said, “it will be in the future, when research has penetrated far deeper into the riddle of the properties of matter.”
The century or so of research since Henderson has revealed a compatibility between water and life as we know it that is even more remarkable and intricate than was imagined.1 But it has also shown that the relationship may not be especially exclusive—the strange harmony between life and water may just be another example of the remarkable adaptability made possible by Darwinian evolution.
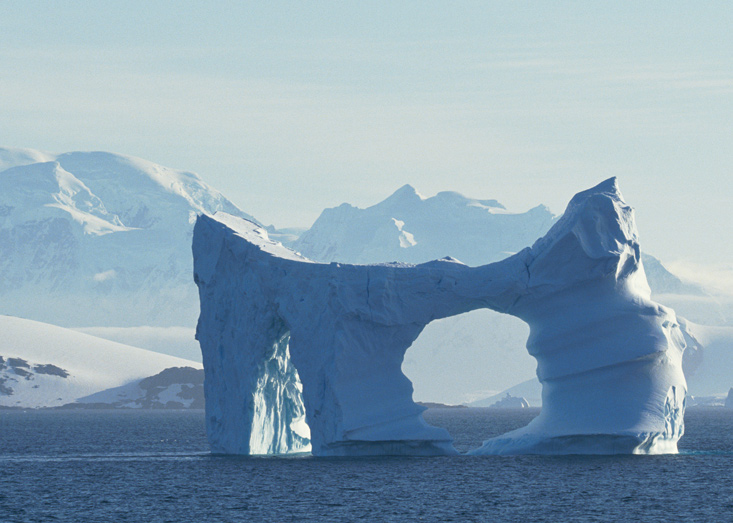
From the modern view, water is far from being a passive backdrop on which life’s biomolecules play out their dramas. It is, instead, an active participant.2 A delicate web of weak chemical bonds between water molecules, called hydrogen bonds, is draped around those biomolecules in ways that weave them into the tapestry of the liquid. This unites the biomolecular solute with its solvent in a mutually responsive dance. To do their job of catalyzing biochemical reactions, proteins have to be rather flexible, changing shape as they guide a reaction along the right path. But these shape-changes remold the surrounding shell of water too, while the wobbles and fluctuations of the water “inject” dynamism into the protein.
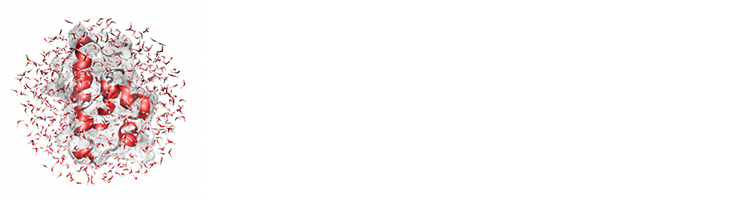
Such interplay can be astonishingly subtle. For example, researchers at the Ruhr University of Bochum in Germany and the Weizmann Institute of Science in Israel have found that, as a protein binds its target molecule (called its substrate) in preparation for transforming it, the water molecules close to the binding site seem to slow down, almost as if thickening to hold the substrate in place.3 And there are subtle gains and losses of energy and entropy, connected to changes in the amount of hydrogen bonding and to the freedom of the water molecules to move around, which can dictate and drive a number of exquisitely tuned and highly selective biochemical processes.
These include the binding of an enzyme with its target molecule or substrate, when water gets expelled from nooks and crannies to make room for the substrate; the folding of a newly made protein chain into the compact shape of an enzyme; the assembly of proteins into multi-part biomolecular machinery; and the assembly of fatty lipid molecules into cell membranes. Each of these processes benefit from the fact that immersion in water somehow induces an attractive force between water-repelling (hydrophobic) parts of molecules.
Water molecules often act like snap-on tools attached to a protein’s surface, thereby extending an enzyme’s reach and helping it bind or transport small molecules. And chains of water molecules threading through channels in enzymes act as “proton wires” that can conduct hydrogen ions, allowing cells to move hydrogen atoms to new locations or a new molecule, or to build up and discharge gradients in hydrogen-ion concentration that can be tapped to generate energy, just as water wheels tap the flow of water down a hillside. Small changes in the web of water molecules threaded amongst the double helix of DNA can affect how easily the molecule bends, and how proteins stick to it to turn the activity of genes on and off.
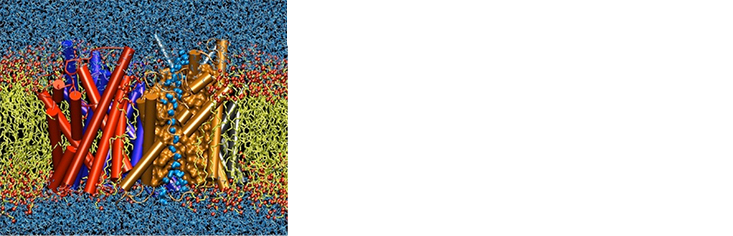
All this shows that water’s role in life is even more complex and comprehensive than Henderson could have appreciated. But how much of it is unique to water, and to what extent does life depend on such abilities? Some of water’s roles, such as in hydrophobic attraction, have analogues in other solvents: If dissolved molecules don’t have much affinity for their solvent, whatever that might be, they’ll tend to stick together. And the conduction of hydrogen ions in water wires, say, is very important for life on Earth, but it’s not obvious that extra-terrestrial biochemistry would find it indispensible.
Another way to pose this question is to ask: What if water were less like water and more like an ordinary liquid? Chemical physicists Ruth Lynden-Bell of Queen’s University Belfast, in Northern Ireland, and Pablo Debenedetti of Princeton University in New Jersey have explored models of “counterfactual water,” in which the feature that lies at the heart of what makes water unusual—its particular hydrogen-bonding arrangement—can be tuned continuously just as if turning a dial. How much tuning is permitted before water’s anomalous nature is lost?
Not all of water’s unique characteristics help life—some are outright hindrances.
One of the simplest theoretical models of water treats hydrogen bonding as purely electrostatic: an attraction between slightly positively charged hydrogen atoms and negatively charged “lone pairs” of electrons on the oxygen atoms of neighboring molecules, with these charges disported at the corners of tetrahedra. This attraction, dictating a particular geometric arrangement of molecules, operates on top of the more general attraction that all atoms and molecules feel for one another owing to the sloppiness of their clouds of electrons, called van der Waals or dispersion forces. In simple liquids such as liquid argon or carbon dioxide, it’s just the van der Waals forces that keep the molecules from flying apart into vapor.
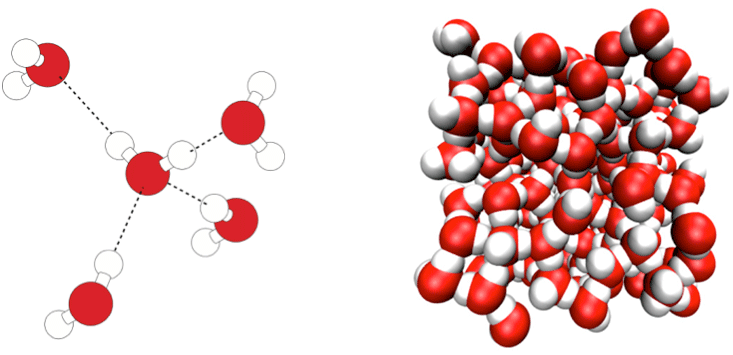
Lynden-Bell and Debenedetti devised a computer model of water in which the relative strengths of the electrostatic hydrogen bonding (which promote tetrahedral ordering) and the van der Waals attractions (which are the same in all directions) could be varied at will.4 Informally, they called this hypothetical stuff “not-water.” What they found was that water-like anomalies are not really just a matter of degree. Rather, the sort of ordering that comes from hydrogen bonding, and the sort that comes from van der Waals attractions of simple spheres, thanks to the way they pack like cannonballs, are distinct and incompatible. In between these two extremes, you get the worst of both worlds: The molecules are less ordered than at either extreme. Water is, in other words, qualitatively different from a liquid that lacks hydrogen bonding, in which the molecules just bump up against each other. Water isn’t, however, the only molecule to make hydrogen bonds—ammonia and even hydrogen chloride can do it, too. The difference is that water molecules can form vast three-dimensional networks because of their tetrahedral pattern of sticking together, while other hydrogen-bonders can only manage chains. The three-dimensional hydrogen-bonded network is the reason why frozen water is less dense than liquid water, something not true for ammonia and hydrogen chloride. Score one for water being unique.
But what about geometry? If you altered the angle of the bent H2O molecule, so that its hydrogen bonds weren’t so nearly tetrahedral, or if you made the bonds longer, would water’s unique properties disappear? When they tried doing this with “not-water,” Lynden-Bell and Debenedetti found that they could still get anomalies such as a maximum liquid density before the freezing point, so long as the changes weren’t too big—for example, so that the water molecule wasn’t too sharply bent. “There is a fair degree of latitude in the [geometrical] parameters,” says Lynden-Bell. The precise degree of variation allowed before a specific property vanishes “depends on which water property one is looking at,” says Debenedetti.
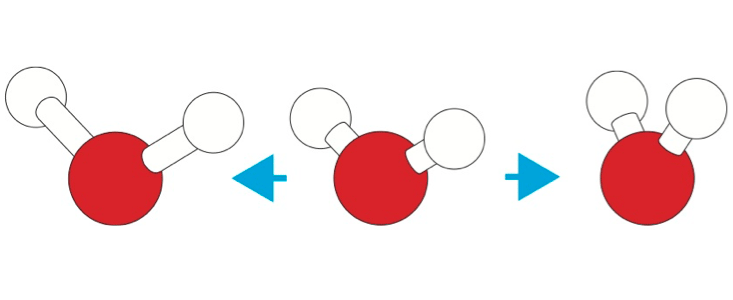
Water’s hydrogen-bonded structure is also often invoked to explain the hydrophobic attraction of solutes it carries. But after looking at “not-water” with differing hydrogen-bond strengths and bond angles, Lynden-Bell, Debenedetti, and their coworkers concluded that the main reason why hydrophobic particles are pretty insoluble in water (and so tend to clump together) is because water molecules are so small that it takes a lot of energy to “scoop out” a space for the particles, and not because of the hydrogen bonding itself.5 So pretty much any liquid with small molecules should show something similar.
On balance, then, water is unique in some ways but not others: special, but not that special. Plus, not all of its unique characteristics help life—some are outright hindrances. For one thing, it’s quite reactive. The lone pairs of electrons on the oxygen atoms are drawn to parts of molecules with a positive charge, where they may break up existing bonds and fragment or reconfigure the molecules in a process called hydrolysis. The carbon atoms in peptide bonds—the linkages that hold together amino acids in protein chains—are susceptible to this sort of attack, giving water a tendency to split proteins through hydrolysis. Water may do the same thing to chains of sugar molecules, like those in biological polysaccharide compounds such as cellulose and starch.
“This is not so much a problem today in living systems, which have enzymes that repair the damage done by water,” says chemist Steven Benner, a distinguished fellow at the Foundation for Applied Molecular Evolution. But it would be important for the origin of life, he says, when proto-biological molecules had to form and persist in water without the help of enzymes. “If water was engineered to be the perfect biosolvent by God, she certainly did a bad job of engineering,” Benner says.
He sees no fundamental reason why solvents such as ammonia, formamide (CHONH2), or liquid hydrocarbons like those on Saturn’s moon Titan shouldn’t also support different kinds of biochemistry.6 After all, a lot of organic chemistry in laboratories and in industry is carried out in non-aqueous solvents (that is, solvents other than water), often precisely because water is too reactive. Benner is particularly interested in the idea that Titan’s hydrocarbon oceans might host hydrophobic life. He and his coworkers recently carried out experiments to see if they could build a “genetic polymer”—one that might encode information in a sequence of molecular building blocks in the way DNA and RNA do—that could work in such a liquid.
They found that chain-like molecules called polyethers, in which carbon and oxygen atoms alternate along the backbone, dissolve pretty well in liquid propane (C3H8) at temperatures of around negative 94 degrees Fahrenheit (negative 70 degrees Celsius).7 Benner argues that polyethers could serve as genetic databases in such a solvent.
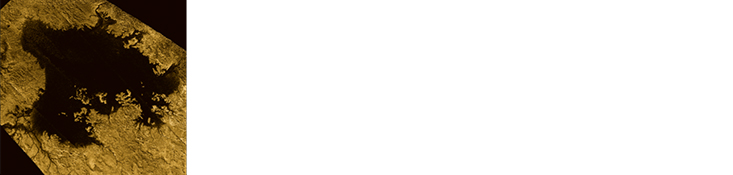
But Titan is much colder than that: Its hydrocarbon oceans, consisting mostly of methane (CH4) and ethane (C2H6), have a temperature of around negative 288 degrees Fahrenheit (negative 178 degrees Celsius). At such frigid extremes, the polyethers don’t dissolve significantly. Benner concludes that liquid methane on Titan is therefore “simply too cold to support the solubility of almost anything necessary to create the properties that we value in life.” But, he says, that’s not because hydrocarbons are bad solvents and water is a good one—it’s just that liquid water is warmer, and stuff dissolves better in warmer liquids. “A warmer Titan,” he says, “with the temperatures in a habitable zone around the sun at approximately the orbital distance of the planet Mars, would have hydrocarbon oceans that include propane, butane, and even pentane.” These solvents would remain liquid at temperatures where they can actually dissolve significant quantities of material.
Another promising alternative to water championed by Benner is formamide, which can be formed from either carbon monoxide and ammonia or hydrogen cyanide and water—all of them simple molecules that can be found in interstellar and extraterrestrial environments. “Formamide is very much like water in terms of its solubility power (possibly even greater) as well as its liquid range (much greater),” says Benner: at one atmosphere pressure, formamide melts at 37 degrees Fahrenheit (2 degrees Celsius) and boils at 411 degrees Fahrenheit (210 degrees Celsius). And it doesn’t have the same tendency as water to bust polymers apart. Benner and coworkers have recently shown that, in the presence of a boron-containing mineral to act as a catalyst, a phosphate chemical group can be added to the molecule adenosine in formamide to make adenosine phosphate, one of the basic building blocks of RNA and DNA.8 In contrast, water tends to split apart adenosine phosphate, making it harder to build nucleic acids.
If it is true that solvents besides water can serve just as well for other life elsewhere in the universe, then the intimate pairing of water and life here on Earth may really be a reflection of the extreme opportunism that adaptation confers on life. Terrestrial organisms use to the full everything that this strange liquid has to offer. It seems ironic that we might be overestimating the importance of water in astrobiology while at the same time underestimating the significance and subtlety of the jobs it does in terrestrial biology.
Indeed, the very adaptability that we find on Earth should give us pause before we elevate water to the sine qua non of life. There are organisms living in cracks in the rock in the hot, pressurized depths of the Earth where oil forms, and within the ice of sub-surface Antarctic lakes. Microbes survive in the baked, dry soil of the Atacama Desert, and whole communities thrive around volcanic hydrothermal vents spewing scalding water in the deep sea. Organisms can survive in extremely salty water, they can tolerate high concentrations of toxic heavy metals and even exposure to the highly ionizing radiation of outer space. While no known organisms can sustain metabolism without at least some water, microbes can adapt to life in heavy water, while some isolated enzymes can work in more or less totally water-free conditions. Given all this, it seems tempting to believe that, once Darwinian evolution gets going, it will find its feet in almost any circumstance.
Lynden-Bell agrees that we shouldn’t underestimate the ability of natural selection to find ways of sustaining life in all kinds of environments. “I personally believe that evolution can exploit the environment that it finds and that it is possible to imagine alternative scenarios,” she says. Life can also change its environment to suit itself. Colin Goldblatt of the University of British Columbia in Vancouver points out that, while cold and moist worlds are the only ones that look habitable from our current perspective, exactly where the zone of habitability lies depends on what else is in the atmosphere: On Earth, carbon dioxide (maintained partly by life) keeps most of our planet above the freezing point.9 In other words, “habitability depends on inhabitance,” he says. To put it another way, we can’t easily judge if a water world could support life without knowing if it already does.
Now, multiply that adaptability by the number of planets that could host it. Judging by the current statistics, just about every star in our galaxy has at least one planet on average, and one in five sun-like stars are likely to have Earth-like planets in their habitable zone. That makes at least 11 billion of these worlds in the Milky Way alone—and there are at least 100 billion galaxies in the observable universe.
In this picture, can we really insist that water be the only solution?
Philip Ball is the author of Invisible: The Dangerous Allure of the Unseen and many books on science and art.
References
1. Lynden-Bell, R.M., Conway Morris, S., Barrow, J.D., Finney, J.L., & Harper, C. Water and Life: The Unique Properties of H2O CRC Press, Boca Raton, Florida (2010).
2. Ball, P. Water as an active constituent in cell biology. Chemical Reviews 108, 74-108 (2007).
3. Grossman, M., et al. Correlated structural kinetics and retarded solvent dynamics at the metalloprotease active site. Nature Structural & Molecular Biology 18, 1102-1108 (2011).
4. Lynden-Bell, R.M. & Debenedetti, P.G. Computational investigation of order, structure, and dynamics in modified water models. Journal of Physical Chemistry B 109, 6527-6534 (2005).
5. Lynden-Bell, R.M., Giovambattista, N., Debenedetti, P.G., Head-Gordon, T., & Rossky, P.J. Hydrogen bond strength and network structure effects on hydration of non-polar molecules. Physical Chemistry Chemical Physics 13, 2748-2757 (2011).
6. Benner, S.A., Ricardo, A., & Carrigan, M.A. Is there a common chemical model for life in the universe? Current Opinion in Chemical Biology 8, 672-689 (2004).
7. McLendon, C., Opalko, F.J., Illangkoon, H.I., & Benner, S.A. Solubility of polyethers in hydrocarbons at low temperatures. A model for potential genetic backbones on warm titans. Astrobiology 15, 200-206 (2015).
8. Furukawa, Y., Kim, H.J., Hutter, D., & Benner, S.A. Abiotic regioselective phosphorylation of adenosine with borate in formamide. Astrobiology 15, 259-267 (2015).
9. Goldblatt, C. Habitability of waterworlds: runaway greenhouses, atmospheric expansion and multiple climate states of pure water atmospheres. Astrobiology 15, 362-370 (2015).
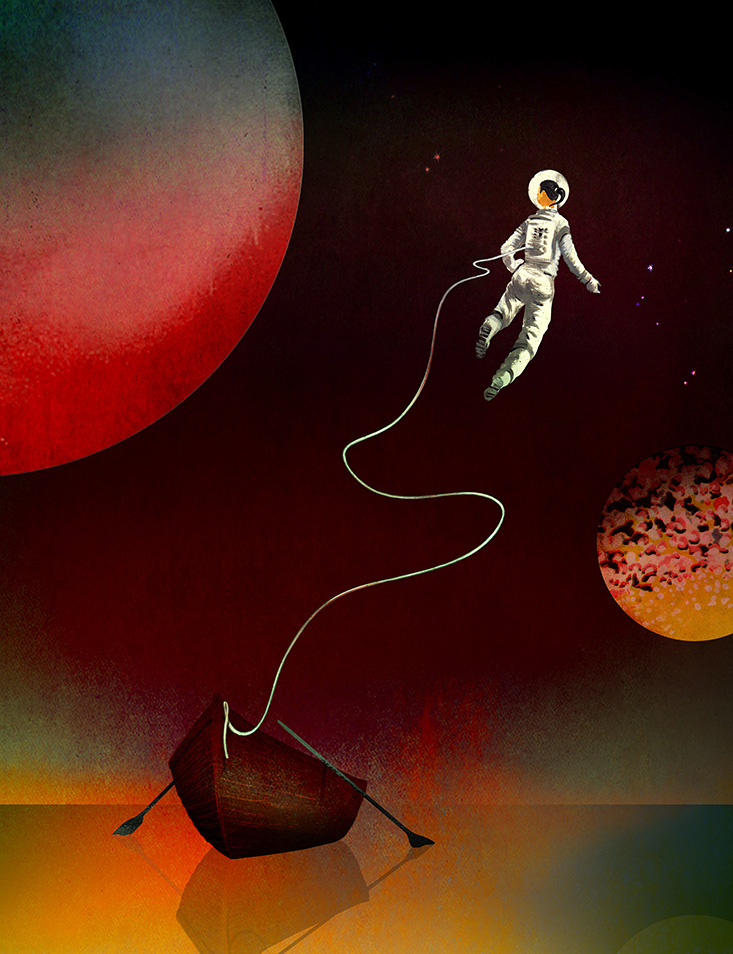