On December 31, 2019, the Chinese government informed the World Health Organization about a cluster of cases, in the city of Wuhan, of a pneumonia of unknown cause.
Less than a year later, on December 8, 2020, 90-year-old Maggie Keenan in Coventry, England, received the first dose of an approved vaccine against that unknown pneumonia, which by then was called COVID-19. In the 11 months between those two events, SARS-CoV-2, the novel coronavirus that causes COVID-19, infected over 68 million people worldwide and killed over a million and a half of them—and the toll continued to soar. Before COVID-19, vaccine development often took decades. The shortest —for mumps—was four years: In 1963, Merck vaccinologist Maurice Hilleman, PhD, took virus samples from his ill five-year-old daughter Jeryl Lynn and used the samples to develop the “Jeryl Lynn Mumps Vaccine,” which won approval from the U.S. Food and Drug Administration (FDA) in 1967.
That first COVID-19 vaccine “will go down in history as one of science and medical research’s greatest achievements—perhaps the most impressive,” declares Eric Topol, MD, the director of the Scripps Research Translational Institute. And several other vaccine candidates were close on its heels.
A novel pathogen had threatened lives and economies worldwide, but within months science had produced vaccines with the potential to stop it. As with so many successes in science, the COVID-19 vaccine resulted from numerous prior achievements (and failures), often in seemingly unrelated areas of study—combined with a bit of good luck.
The history of vaccinology begins long before humans had any knowledge of pathogens or of the immune system’s protective effects.
“The success of the COVID vaccines was only made possible by decades of basic science research,” says Yale immunologist Akiko Iwasaki, PhD.
Many of the innovations most crucial in developing the new vaccines occurred quite recently. “If COVID had come along a decade ago or 15 years ago, we’d be in a lot more trouble than we are right now,” says Wayne Koff, PhD, the president and CEO of the Human Vaccines Project.
But could science have gone even faster? And in the future, could we do a better job developing vaccines—and generating fundamental understanding of human biology—to combat not just future novel pathogens but many chronic health hazards?
“There is no question we can and should get a whole lot better at making vaccines faster, with a wider range of targets,” Koff says.
Starting With Scabs
Vaccination is one of public health’s greatest achievements, responsible for saving many millions of lives. That is why government research agencies—not to mention Wellcome, the Gates Foundation, and other philanthropic organizations—have devoted so much support to developing and distributing vaccines. The goal of almost all vaccines is to coax the body’s immune system into resisting pathogens. Until well into the 20th century, however, scientists produced vaccines as a hit or miss proposition, with little knowledge of why they worked or failed.
The history of vaccinology begins long before humans had any knowledge of pathogens or of the immune system’s protective effects. By the 16th century, historical records indicate that Chinese practitioners were inserting scabs from smallpox victims into the noses of healthy people. This procedure, called variolation, proved effective at preventing smallpox by causing a mild case—though up to 3% of people got such a severe case of smallpox they died. Still, many found that risk acceptable.
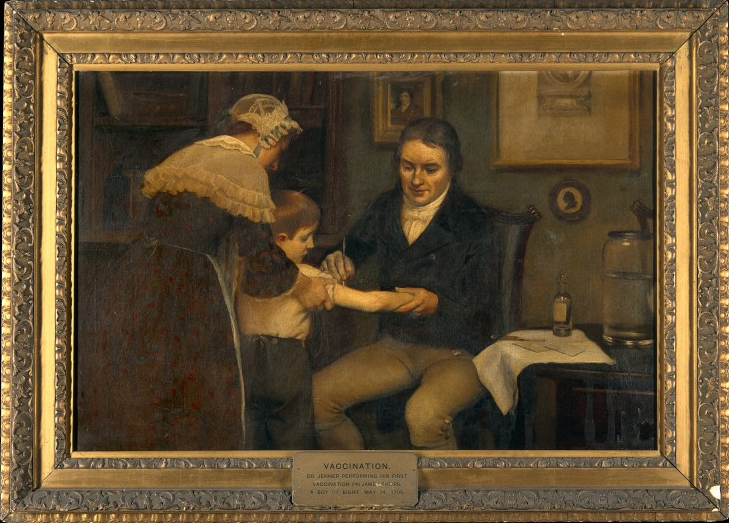
Lady Mary Wortley Montagu, wife of the British ambassador to the Ottoman Empire, witnessed variolation there and in 1721 brought word of the procedure home. There, it gained increasing acceptance. Some decades later, Edward Jenner, a country surgeon, observed that milkmaids who got a rare and fairly harmless disease called cowpox seemed to be immune to smallpox. On May 14, 1796, he infected 8-year-old James Phipps with cowpox, then in July infected Phipps with a full dose of smallpox. The boy developed no serious disease. Over the next few years, Jenner repeated the experiment in a handful of other people and published his results. Though the concept met some initial resistance, vaccination (a word derived from vacca, Latin for cow) gained widespread acceptance in the early 19th century.
In the latter part of the 19th century, French chemist Louis Pasteur, German physician Robert Koch, and several contemporaries discovered the bacteria that cause cholera, plague, and anthrax. They learned to grow the organisms into strains that did not cause disease (a process called attenuation) and experimented with either attenuated or killed organisms to vaccinate humans and animals. In 1890, Emil von Behring, MD, and Kitasato Shibasaburō, MD, of Koch’s lab published their discovery that serum from animals who had suffered from diphtheria or tetanus contained molecular compounds that neutralized the toxins released by the bacteria. German physician Paul Ehrlich, MD, whose work on immunity earned him the Nobel Prize, called these molecules “antibodies.” And Élie Metchnikoff, PhD, cowinner of the Nobel with Ehrlich, had a few years earlier identified white blood cells’ ability to gobble up bacteria and other foreign bodies.
Building on a Handful of Successes
At the beginning of the 20th century, just five vaccines were used by doctors to prevent human disease: live-virus smallpox and rabies vaccines, and vaccines employing killed bacteria against typhoid, cholera, and plague. In addition, immunization for diphtheria or tetanus became widely accepted medical practice. With a few exceptions, not much else happened in vaccinology for another 50 years.
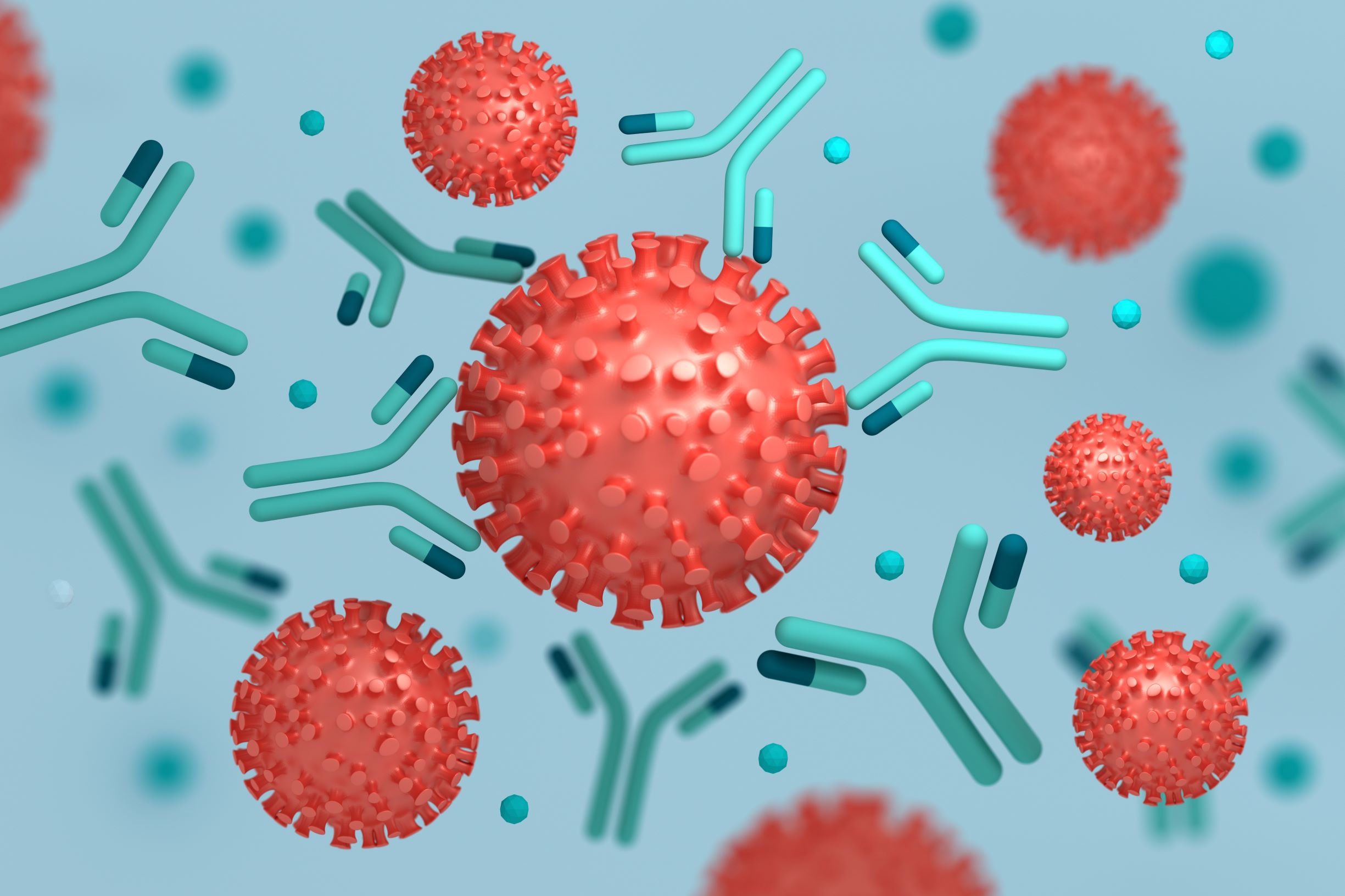
Vaccine production accelerated just before and after World War II, as scientists developed the technology to grow organisms in cell cultures in quantities large enough that the organisms could be killed or attenuated and used as vaccines. In 1931, pathologist Ernest Goodpasture, MD, and virologist Alice Miles Woodruff, PhD, of Vanderbilt discovered that they could grow large amounts of virus on the outer membrane of the eggs of fertilized hens. This technique is still used today to produce several vaccines, most notably the one against seasonal influenza. Another big advance was growing viruses in laboratory cultures of cells—a process that could be scaled up to industrial levels. Hugh Maitland, MD, and Mary Cowan Maitland, MD, of the University of Manchester in England, accomplished this first, in 1928, with vaccinia, the virus that causes cowpox, growing it in chicken kidney cells. In the late 1940s, John Enders, PhD; Thomas Weller, MD; and Frederick Robbins, MD, of Boston Children’s Hospital grew poliovirus in culture. This work, for which they won the 1954 Nobel Prize, led to the Salk and Sabin polio vaccines.
Research with killed or attenuated viruses has also yielded vaccines against nearly 20 other diseases, including smallpox, cholera, tuberculosis, measles, mumps, and rubella.
Since 1920, scientists have also augmented vaccines with seemingly unrelated chemicals known as adjuvants, which boost the immune system’s response to the vaccine and so allow for smaller or fewer doses. As is the case with many scientific findings, fortuity played a role in the discovery of adjuvants. Gaston Ramon, a French veterinarian, was working for the Institut Pasteur on vaccines against tetanus and diphtheria. The process involved injecting horses with tetanus or diphtheria toxin, collecting blood samples from the infected horses, and using the antibody-rich serum to inoculate humans. When Ramon realized he was able to harvest more antiserum from horses that exhibited inflammation at the injection site, he tried injecting starches along with toxins and found if he thus elicited inflammation intentionally, he could increase the production of antiserum. Adjuvants used today include aluminum salts, oil-in-water emulsions, and other organic and inorganic chemicals. The mechanism of many adjuvants remains unclear and is the subject of intense research. In most cases, they enhance, allow, and create the nonspecific or innate portion of the immune system’s reaction, allowing for a better response from the adaptive part of the immune system and creating longer-lasting immunity.
In the late 1970s, the advent of recombinant DNA—DNA from different species of organisms—allowed scientists to create vaccine candidates that introduced to the body pieces of viruses or substances called virus-like particles. They often introduced viral genes into vectors, such as a harmless virus that could carry the possibly immune-inducing substance into the body. The first major success was the hepatitis B vaccine, licensed in 1986.
To this day, more than 35 years later, there is no successful HIV vaccine—but without the search for one, it is unlikely we would have had vaccines against COVID-19 so quickly.
Meanwhile, also during the latter part of 20th century, scientists discovered more details regarding the astounding complexity of the immune system. But until very recently, vaccinologists did not develop vaccine candidates based on this knowledge. Almost every vaccine was a hit or miss proposition. Scientists could try to determine why they failed or succeeded. But vaccinology and immunology remained virtually separate fields.
Failure Breeds Eventual Success
A major impetus for change came from the expensive, massive failure to develop a vaccine against the human immunodeficiency virus (HIV), which causes AIDS. On April 23 1984, Margaret Hecker, the secretary of the U.S. Department of Health and Human Services, announced that scientists at the National Cancer Institute had isolated HIV. (Ultimately, much of the scientific world, including the Nobel Prize Committee, concluded that the French had discovered the virus first.) Heckler declared correctly that the discovery of the virus would lead rapidly to a blood test to detect it. She also predicted that “yet another terrible disease is about to yield to patience, persistence, and outright genius,” saying there would be a vaccine within two years. Many regard Heckler’s conceit about the power of vaccinology as a prime example of hubris. To this day, more than 35 years later, there is no successful HIV vaccine—but without the search for one, it is unlikely we would have had vaccines against COVID-19 so quickly.
“They’ve made huge advances in understanding vaccine immunology and how to do human clinical trials quickly for vaccines, including having all kinds of details in place. All that infrastructure that was able to be employed within days of having the SARS-CoV-2 genome was developed by the HIV projects.”
Governments and private donors have spent roughly $20 billion to date on the search for an HIV vaccine, but every one of the 200 or so candidates has failed either in clinical trials or before making it that far. HIV is a pathogen that is especially resistant to a vaccine. Upon entering a cell, it immediately coats itself with a polysaccharide, a sugar, which covers the proteins that might elicit an immune response. In addition, HIV targets particular T cells known as CD4s, often described as the master controllers of the immune system. A common metaphor casts HIV as an arsonist that destroys firehouses before attacking other structures.
But the societal need for an HIV vaccine is such that armies of scientists kept going back to the drawing board—looking at immune system basics, searching for ever more potential vaccines, and setting up complex clinical trials networks to study them.
“The HIV efforts contributed a lot to the success COVID-19,” says Shane Crotty, PhD, of the La Jolla Institute for Immunology. “There was enough of a push in the HIV world to measure all kinds of things in the immune system that otherwise weren’t being measured. There were a whole bunch of labs that were working on HIV that immediately pivoted to COVID-19 and did very high-level work because they had developed the necessary tools.”
Crotty points out that many HIV vaccine research projects have been criticized over the years for their expense and lack of success, but he feels the COVID-19 effort has more than vindicated them. “They’ve made huge advances in understanding vaccine immunology and how to do human clinical trials quickly for vaccines, including having all kinds of details in place. All that infrastructure that was able to be employed within days of having the SARS-CoV-2 genome was developed by the HIV projects,” Crotty says.
Taking Aim at SARS-CoV-2’s Spikes
As soon as Chinese scientists posted SARS-CoV-2’s genetic sequence, on January 10, 2020, researchers around the world realized they were not confronting a challenge with HIV’s complexities. A large protein, called a spike or S protein, protrudes from SARS-CoV-2 and is critical to its ability to enter a variety of human cells. It was the obvious candidate for a vaccine.
The NIH’s Center for Vaccine Research was originally established to coordinate the search for an HIV vaccine, but it soon turned to address various pandemic threats. As part of that effort, NIH researchers had been studying the spike protein on the virus that causes Middle East respiratory syndrome (MERS), a potentially lethal cousin of COVID-19 that people can catch from camels. In their work with MERS, the scientists discovered that the spike protein exists in two forms—“prefusion,” which induces immunity, and “postfusion,” which doesn’t. They also discovered how to genetically engineer the protein to keep it in the desired form. This turned out to be a relatively simple task because so much about the genetics of protein structure was understood from research performed first for HIV, then for MERS and other viruses.
To most of the public, the concept of using mRNA to deliver a vaccine seemed to come out of nowhere. In fact, it resulted from almost two decades of difficult and often failed basic science research, injecting cells first with DNA and then with mRNA.
“Because of our earlier work with MERS, we were able to have the correct form of SARS-CoV-2 ready for experiments in a few days,” says Kizzmekia Corbett, PhD, a researcher in the NIH vaccine group. To employ an immune response against the spike protein, the NIH scientists did not inject it directly into the body. Instead, working with the biotechnology company Moderna, they injected a modified form of messenger RNA (mRNA), the genetic instructions directing cells to make spike proteins. This turns cells in the body into minifactories churning out spike proteins, which sets off a protective immune response. The pharmaceutical giant Pfizer, together with the biotechnology company BioNTech, employed an almost identical strategy. Both mRNA-based COVID-19 vaccines won Emergency Use Authorization from the FDA in December 2020.
To most of the public, the concept of using mRNA to deliver a vaccine seemed to come out of nowhere. In fact, it resulted from almost two decades of difficult and often failed basic science research, injecting cells first with DNA and then with mRNA. As scientists learned increasing amounts about cell biology, many hypothesized that simply injecting genetic information might solve many problems. It could instruct cells to produce proteins that treat disease or proteins that act as a vaccine and stimulate an immune response. Dreaming up such ideas is simple. Executing them is a gargantuan task. If a simple molecule of DNA or mRNA is injected into the body, the innate immune system instantly destroys it before it can give any orders.
Harnessing the Power of Messenger RNA
For much of the early 1990s, biochemist Katalin Karikó, PhD, was viewed as a failure. Born in Hungary and working at the University of Pennsylvania, she strongly believed in the power of artificial mRNA to produce proteins on demand. But one experiment after another flopped. She could not get funding. The university kicked her off the tenure track and demoted her. “Every night I was working—grant, grant, grant,” Karikó told the online publication STAT. “And it came back always no, no, no.”
Such stories are depressingly common in science. A major criticism of the U.S. government’s funding agencies, such as the NIH, is that they often support projects so far along that success is almost guaranteed.
But despite the pressure, Karikó persisted. Together with a Penn colleague, Drew Weissman, MD, PhD, she figured out how to put artificial building blocks known as nucleosides into the mRNA and coat the molecules with nanoparticles of lipids (fats). Karikó and Weissman’s ideas caught the attention of BioNTech in Germany and Moderna in Cambridge, Mass., positioning them to produce and test the successful mRNA vaccines against COVID-19—the first using mRNA technology.
Many believe that vaccinology’s future—the development of still more and better vaccines—lies in an even better understanding of the immune system. Despite the many lives saved to date by vaccines, the list of remaining vaccinology challenges is longer (and the catalog of approved vaccines shorter) than most people realize.
In recent years, the NIH had tried using mRNA to deliver candidate vaccines against Zika and Ebola. These stand out as the first examples of knowledge about immunology being applied to vaccinology. But these experiments went on the back burner as the threats from those viruses appeared to subside.
Other COVID-19 vaccine candidates that have entered Phase 3 clinical trials are delivered to the spike protein by methods (called platforms) other than mRNA. A common one is to fuse genes from the S protein into a harmless virus, an adenovirus similar to those that cause the common cold. This technology was developed almost entirely as part of the effort to find an HIV vaccine. Another platform injects a modified version of the S protein directly into the body.
Many believe that vaccinology’s future—the development of still more and better vaccines—lies in an even better understanding of the immune system. Despite the many lives saved to date by vaccines, the list of remaining vaccinology challenges is longer (and the catalog of approved vaccines shorter) than most people realize. HIV is far from the only failure to date. Despite decades of trying, for example, scientists have yet to win approval for a vaccine against respiratory syncytial virus—a major killer of young children. And cytomegalovirus infects most people, usually without ill effect; but it can be dangerous to young children, immunocompromised people, and the elderly, yet there is no vaccine against it. And few vaccines exist for bacterial or parasitic diseases, despite the enormous worldwide burden they represent. Many see promise in the technique of reverse genetic engineering to evoke immune responses to key parts of these relatively large organisms—bacteria and parasites being much bigger than viruses.
Merging Vaccinology and Immunology
With a better understanding of the immune system, it could be possible to shorten the time between identification of a pathogen and development of a vaccine to even less than the speedy 11 months that it took in the case of COVID-19. For example, clinical trials might be shortened considerably if we recognized changes in the immune system that serve as markers for a successful vaccine.
Before COVID-19 struck, most scientists expected the next pandemic threat to emerge from a new strain of influenza. It is widely understood that the constant mutations of influenza viruses require a new flu vaccine every year. And scientists have constantly worried that inevitably an influenza virus mutation will bring a repeat of the 1918 pandemic. Though several research groups are working on a universal influenza vaccine, one that would protect against any mutation that might come along, their progress so far is nowhere near their hopes.
“The more we understand about basic biology, the more we understand about the immune system. You can’t separate the two.”
It’s not just infectious diseases where a better understanding of the immune system holds promise. “If you ask where you could you put in a major influx of resources and institutions and make a big contribution, human immunology is that area,” says Nobel Laureate David Baltimore, PhD, the president emeritus of Caltech and a senior science advisor for the Science Philanthropy Alliance.
The key, Baltimore and others say, is learning more about inflammation—a protective response by the immune system to cellular damage, pathogens, or other harmful stimuli. Although inflammation is often a normal, healing reaction to irritants like a wound, a foreign body, or a virus, inflammatory reactions can also get out of control in conditions like cancer, heart disease, or Alzheimer’s. Many aspects of the aging process also seem tied to inflammation.
There have been many attempts to create vaccines to treat or prevent cancer, and work is ongoing on vaccines against heart disease and Alzheimer’s—but progress is slow.
“The absolutely outstanding question is the control of inflammation and its role in aging and its role in diseases that are linked to aging,” says Baltimore. “We just haven’t had a way of getting into it. The more we understand about basic biology, the more we understand about the immune system. You can’t separate the two.”
“My hope is that this success will propel even better forms of vaccines to be developed against other infectious diseases and noninfectious diseases like cancer,” echoes Iwasaki.
A chorus of scientists agree that the key to better health—in response to both emerging threats like COVID-19 and major chronic killers—lies in better understanding the immune system. A major question is whether society will commit to that goal resources even close to those that fueled the COVID-19 vaccine success story.