It may seem absurd to compare a tiny fruit fly’s brain to that of a majestic elephant. Yet it is the dream of many neuroscientists to find deep rules that very different brains share. As Gilles Laurent, a neuroscientist at the Max Planck Institute for Brain Research in Frankfurt, Germany, who has studied a variety of animals, from locusts to turtles, has said, “Neural responses can be described by the same mathematical operation … in completely different systems.” Vivek Jayaraman, a researcher at the Howard Hughes Medical Institute’s Janelia Research Campus, and a former student of Laurent’s, believes that neuroscientists are on the verge of identifying some of these deep neural rules. Grasping them would advance another neuroscientific dream: to be able to predict animal behavior as easily as Newton could predict the behavior of a moving object.
Jayaraman and a small number of researchers studying the brain’s GPS have, in fact, already experienced the thrill of discovering one such rule. It governs something essential—the ability of an animal to keep track of where it’s headed. What’s more, recent experiments on flies hooked up to virtual-reality environments—one from Jayaraman’s group and another from Rachel Wilson (a former postdoc of Laurent’s) and colleagues at Harvard—show how a fruit fly’s visual cues ensure the stability of its heading. The findings offer insight into how mammals, like us, might build maps of their world.
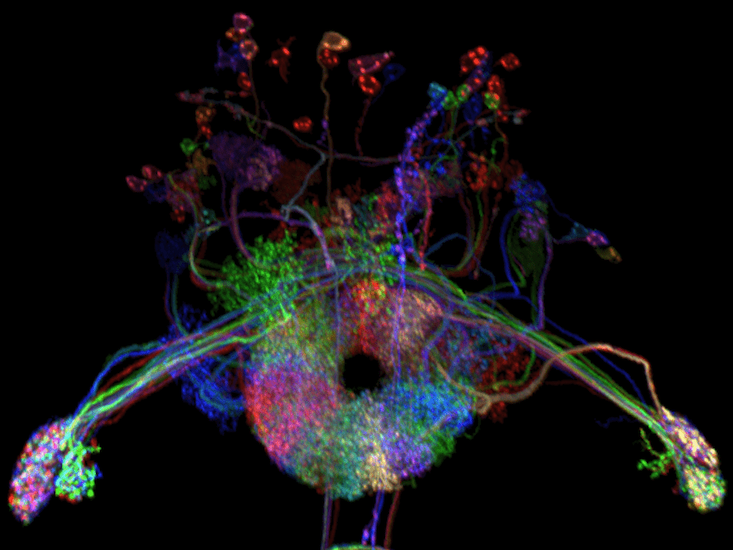
When you’re moving freely, certain neurons are only active when you’re facing a particular way. It does not matter where you are—as long as you keep looking ahead, in that direction, the cell fires. These so-called head-direction cells were first identified in a region of the rat brain very close, and intimately linked to, the hippocampus, the home of place cells, and the basis for your map of the world. There are a large number of these cells, but any one cell is only active in a certain small region of the environment. They act like a map, telling you where you are. Such a map isn’t very useful without knowing where you’re headed. That’s why the brain also creates a mental compass, using head-direction, or compass, cells.
It is striking how closely these two systems work together. If you were to rotate the visual cues that a rat uses to define its heading, then the head-direction cells would remap themselves to this shifted world. At the same time, the place cells of the hippocampus would also rotate the mental map. These two groups of cells provide complementary information that, if correctly put together, are enough to help the rat navigate anywhere in its environment—even in the dark. Head-direction cells, in other words, don’t simply respond to visual cues. They maintain their activity even when a rat can’t see its surroundings (or when you cover your eyes). How does the brain set up this stable compass-like representation? More perplexingly, how does it maintain this stable heading without visual cues?
Scientists can predict whether a fruit fly thinks it is turning right or left.
Experimental evidence in rats and mice suggests that a doughnut-like structure of head-direction cells keeps the brain’s compass reliable. A 1995 paper, “A model of the neural basis of the rat’s sense of direction,” first proposed this doughnut or “ring-attractor” picture. In it, William Skaggs and his colleagues from the University of Arizona hypothesized that the head-direction cells—which did not seem to have any visible characteristic group structure—connect together to form an imaginary ring that creates a 360-degree map of two-dimensional space. “The aim of this effort is to develop the simplest possible architecture consistent with the available data,” the researchers wrote. “The reality is sure to be more complicated than this model.”
Each cell, a point on the ring, refers to a particular heading. Nearby cells on this ring refer to adjacent and similar headings, and activate each other, while cells that are far apart, and refer to opposing or near-opposing headings, deactivate each other. The researchers suggested a rule for how information from the brain’s visual areas enters the ring of head-direction cells, contributing to their characteristic activity pattern. They also incorporated the vestibular system of the brain, which detects head turns, to allow the ring to maintain its activity in the dark. This model of inputs allowed for only one small group of nearby head-direction cells to be active at any given time. This one “bump” of activity corresponded with the rat’s heading. If the rat, or its world, turned (by, say, the rat getting flipped over) then the connections within the ring of cells and the changing visual input information would cause the bump to also turn accordingly.
In a win for Skaggs and his colleagues, researchers have identified some of the cells that appear to operate according to their mathematical model. Still, it has one major hole: There is little understanding of how the brain converts visual information into a stable sense for heading. It is, at least for now, too hard to map how the millions of cells and complex connections that allow rats and mice to see connects to this ring.
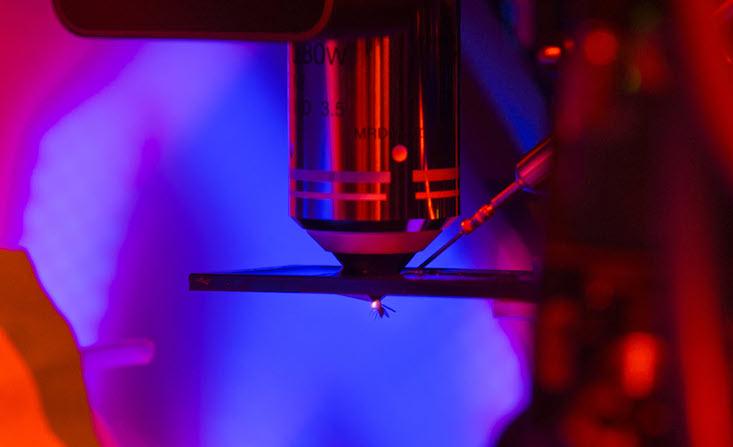
This is where Jayaraman comes in. The fruit fly, it turns out, also has a region of its brain dedicated to maintaining a sense of direction. Jayaraman characterized it with his colleagues less than a decade ago. Incredibly, it is a doughnut-shaped ring exactly like the structure of the theoretical ring-attractor network. Any given cell in this ring of cells is only active when the fly is facing a particular way—exactly like head-direction cells in rodents (and, presumably, in us). This discovery provides a terrific opportunity, not only to test a model that scientists developed for one animal using a completely different animal, but also to identify a common rule that a striking variety of brains use.
“Our paper provides evidence to support the model put forward by Skaggs et al.,” Yvette Fisher, the lead author, told me. “Particularly the requirement by the model that visual inputs to the compass neurons are modifiable.” The two studies, in other words, identified a rule, or mechanism, that dictates how the strength of connections between neurons in the ring and visual inputs change. The ring’s visual inputs inhibit head-direction cell activity—the head-direction cell with the weakest connection to the visual input neurons will be active.
For Fisher, who is a neurobiologist, being steeped in an evolutionary mindset might have shaped her expectations. “It isn’t surprising that common principles exist when the neural circuit required is under similar constraints,” Fisher said. “Navigation has a number of broadly similar requirements for all animals.” Given these similarities, the rules the researchers identified could inspire others to make sense of this process in mammals. General principles, like the ring-attractor model for calculating heading, provide insight into how all animals behave. Scientists can use them to predict some animal behavior, like whether a fruit fly thinks it is turning right or left.
What’s more, scientists now have a baseline model from which they can see how specific animals deviate from one another. For example, while a rat, a terrestrial animal, might make do with a two-dimensional model of heading, birds and bats and some insects fly. While much of the work on flies has focused on walking flies, recent research on bats has found a three-dimensional map.
It is still an open question whether the same neural algorithm expands from two to three dimensions in these animals. Potentially even more interesting is how some animals navigate over long distances: Desert ants are known to be able to locate their nest, a three-dimensional tunnel structure, from miles away, and many migrating birds can return to the same location year after year. Fisher believes that the answers will come from characterizing a diversity of brains, and comparing the algorithms each creature evolved.
Adithya Rajagopalan is a third-year graduate student in the department of neuroscience at Johns Hopkins University & Janelia Research Campus. Follow him on Twitter @adi_e_r.
Lead image: Herbert Kratky / Shutterstock