Hot fluids of neutrons that flow without friction, superconductors made of protons, and a solid crust built of exotic atoms—features like these make neutron stars some of the strangest objects we’ve found in the cosmos so far. They pack all the mass of a star into a sphere the size of a city, resulting in states of matter we just don’t have on Earth.
And yet, despite their extreme weirdness, neutron stars contain a mishmash of vaguely familiar features, as if seen darkly through a funhouse mirror. One of the weirdest is the fact that deep inside a neutron star you can find a whole menu full of (nuclear) pasta.
The forces and densities couldn’t be more different, yet the shapes that emerge are amazingly similar.
The pasta is made of protons and neutrons, held together by the extreme pressures. These oddball nuclei arrange themselves into weird configurations that Matt Caplan of Indiana University and his colleagues call “nuclear pasta.”1 The pasta layer lies in the inner crust, a transitional zone between a neutron star’s outer crust and core. In the top of this layer, the nuclei form blobs called “gnocchi.” Deeper down, they join together into cylindrical shapes called “spaghetti.” More pressure, and the spaghetti compresses into “lasagna”: flattish sheets of nuclear matter. Then the pasta transitions into “anti-pasta”: The sheets of lasagna form cylindrical hollows where neutrons begin leaking out, which Caplan calls “anti-spaghetti.” And finally, when the pressure is high enough, those hollows break into small bubbles, the “anti-gnocchi” phase.
And yes, these are all terms used in papers published by Caplan and his team. One paper even makes reference to “nuclear waffles,” which are like lasagna with holes.2
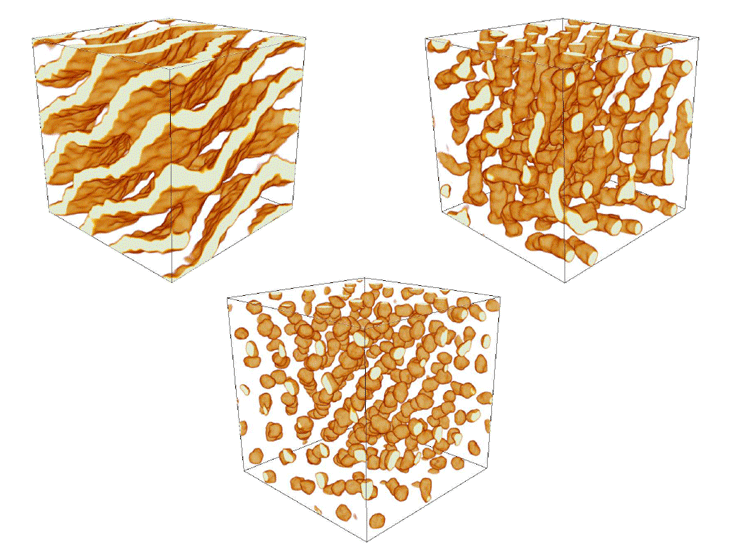
Weirder still is the resemblance of the nuclear pasta to structures formed by certain biological molecules. These molecules are lipid polymers, which are found in fats. Because they are made of a water-loving and water-repelling layer sandwiched together, their interactions with a watery environment make them self-assemble into the spaghetti- and lasagna-like structures known as endoplasmic reticulum, found in complex (eukaryotic) cells.
The similarity is striking, even though the systems couldn’t appear more different in most respects. Nuclear pasta is roughly 100 trillion times denser than the interior of a cell—that’s 1014, or a 1 followed by 14 zeroes. The forces in a neutron star are strong electromagnetism and the nuclear forces; cells are governed by weaker molecular electric forces and the microscopic properties of water. Even the building blocks are different: The raw materials for nuclear pasta are protons and neutrons, while endoplasmic reticulum is made of long chains of molecules strung together.
Caplan says, “What’s in common is that they want to minimize surface energy.” Think of a soap bubble or a splash of water aboard the International Space Station: They form roughly spherical shapes. That’s because the surface forces draw the molecules together into spheres, which involve the lowest amount of energy on the surface. The forces that create nuclear pasta and endoplasmic reticulum aren’t as symmetrical, thanks to the competing attractive and repulsive interactions between the lasagna layers and the surrounding material. However, the laws of physics are still at work and want to minimize the energy involved. The result is blobs and folded sheets and cavities in the neutron star crust or the watery interior of a cell. The forces and densities couldn’t be more different, yet the shapes that emerge are amazingly similar.
“You have to understand the crust, otherwise you don’t understand anything.”
Another eerie resemblance explains why scientists are so interested in nuclear pasta to begin with. The pasta layer is sandwiched between the neutron star’s hard outer crust and superfluid interior. Below the anti-gnocchi layer, nearly every proton merges with an electron to make neutrons, which marks the boundary where the inner crust ends. Below that is the neutron star core, which is a neutron superfluid: a liquid that flows without any resistance. (Researchers think there’s an inner core as well, but exactly how that works is a matter of some contention.) Sitting between the freely-flowing neutron fluid and the rigid iron outer crust, nuclear pasta is the interface between very different zones.
This is just like the plastic-like mantle on Earth, which lies between the flowing molten outer core and the solid crust. Earth’s mantle is key to understanding earthquakes, and a neutron star’s pasta layer is essential for describing one of the most dramatic stress releases anywhere in the universe: the starquake.
“If you have a very large magnitude earthquake, seismologists detect both the initial rupture of the crust and the seismic waves bouncing around inside the Earth,” says Anna Watts, a neutron star astronomer at the University of Amsterdam. Similarly, “starquakes become quite an interesting thing, because they effectively let you peer inside the [neutron] star by looking at the response of the star.” Just as earthquakes led researchers to discover the existence and nature of Earth’s core, starquakes vibrate through the entire neutron star interior.3 What happens on the crust doesn’t stay there: A shattering of the solid crystal transmits vibrations through the pasta layer into the core, and in turn can feed back into how the crust behaves. But what we observe—indirectly—is the way the crust fractures and reforms. “When you look at a neutron star, what you see is the crust,” says Caplan. “You have to understand the crust, otherwise you don’t understand anything.”
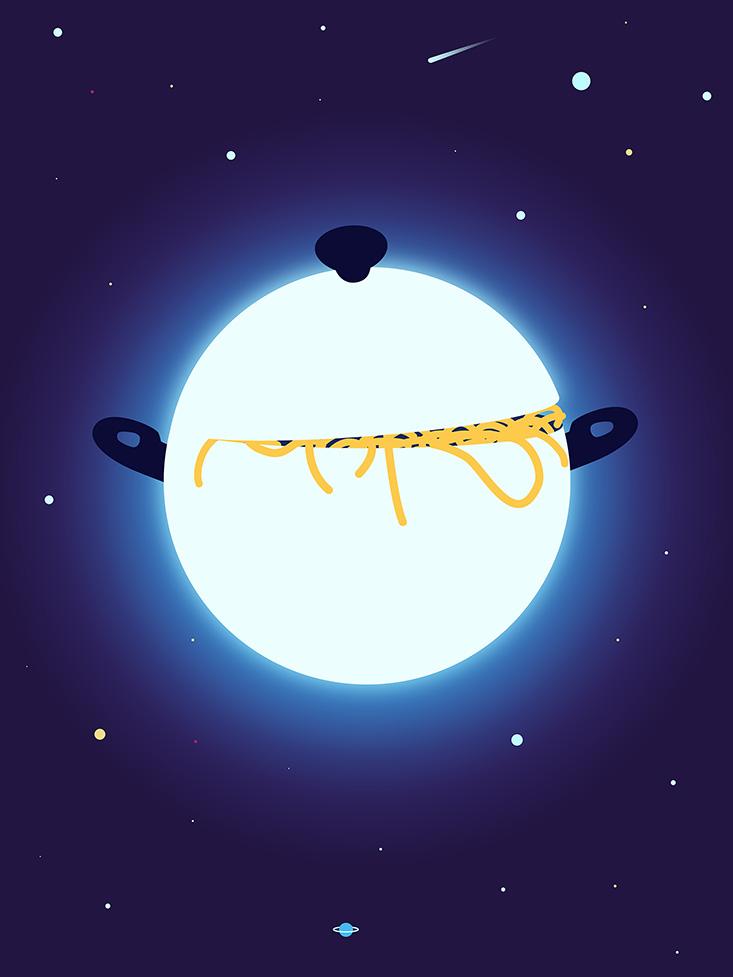
In fact, it’s when a neutron star is undergoing a starquake that it’s most visible from Earth. Neutron stars are too tiny to see directly, but a few have such intense magnetic fields that they are known as magnetars. These are likely among the youngest neutron stars, and their strong magnetic fields produce space weather similar to what we see from the sun during solar storms—though cranked up to 11. Magnetic fields don’t like to be packed tightly together, so they “recombine,” snapping together and releasing a huge amount of energy. The result is the fracturing of the magnetar’s crust, a huge starquake, and a burst of gamma rays.
“The very bright initial flash of gamma rays tends to fry any satellite looking at it immediately,” says Watts—though a telescope doesn’t have to be looking right at a magnetar to see these bursts. Fortunately for satellites, but unfortunately for scientists, gamma ray flares like this are rare: Only three have happened since we’ve had the equipment to see them.
But that’s what we see from the Earth: the gamma rays and other light from the neutron star. Using the magic combination of observation, theory, and computer simulations, we can trace the burst back to the starquake—and the pasta layer between the fractured crust and fluid core.
Understanding nuclear pasta could help us understand not only what’s going on during crust-rupturing starquakes, but reveal some of the mysterious dynamics of the neutron star core. The core of a neutron star is a phenomenal dynamo, producing the most intense magnetic fields we know. These magnetic fields must be made by moving electric charges, but the precise nature of the dynamo is unknown. That’s because nearly everything about the neutron star core is inaccessible to experiments on Earth, thanks to the intense pressures involved. Researchers don’t have a clear theoretical picture connecting the pressure of the core substance to its density and temperature either, which would let us describe all sorts of useful things, like the neutron star size. The churning of material deep in the core is essential for comprehending the entire neutron star, and the pasta layer is the connection between that unknown and the observable properties.
The neutron star is not the first example in nature of a similarity of form across vastly different scales and materials. Unusually persistent waves called solitons are seen in superconducting wires and across oceans, shockwave fronts happen across the light-year expanses of galaxies and in tiny bubbles of water, and vortices characterize everything from tornadoes to magnetic fields. The idea of persistent forms goes back at least to Plato’s Timaeus dialogue, where a demiurge, or divine craftsman, stamps shapes into the material world from eternal and unchanging models. Philosophers and scientists have uncovered a world where the strange echo of the neutron star is not just common, but expected.
Matthew R. Francis is a physicist, science writer, public speaker, and educator.
References
1. Caplan, M.E., Schneider, A.S., Horowitz, C.J., & Berry, D.K. Pasta nucleosynthesis: Molecular dynamics simulations of nuclear statistical equilibrium. Physical Review C 91, 065802 (2015).
2. Schneider, A.S., Berry, D.K., Briggs, C.M., Caplan, M.E., & Horowitz, C.J. Nuclear “waffles.” Physical Review C 90, 055805 (2014).
3. Lander, S.K., Andersson, N., Antonopoulou, D., & Watts, A.L. Magnetically driven crustquakes in neutron stars. Monthly Notices of the Royal Astronomical Society 449, 2047-2058 (2015).
* Image reprinted with permission from Schneider, A.S., Horowitz, C.J., Hughto, J., & Berry, D.K. Nuclear “pasta” formation. Physical Review C 88, 065807 (2013). Copyright 2015 by the American Physical Society.