More than a decade ago, a team of scientists decided they wanted to shoot neutrinos from Fermilab outside Chicago to a target buried in an abandoned gold mine 810 miles away. It was a big idea, one that promised to finally answer decades-old questions about these vexing particles that flood our universe.
It would also take some big components: the most powerful neutrino beams ever created, 10,000 tons of ultra-pure liquid argon, and 800,000 tons of excavated rock. And $1.7 billion, to be provided mostly by the Department of Energy.
Neutrinos have defied definition for nearly 100 years.
After some haggling, the DOE signed on to $850 million, and with some adjustments—and external funding—the epic neutrino experiment now known as DUNE was born in 2015. Or at least began. Since then, there’s been lots of digging, lots of preparations at Fermilab, and lots of refinements in detector technology. But no zapped neutrinos. And now, as DUNE’s scientists ask for more time and more money, the DOE is pausing to ask: How much investment should they—and society—make in big, basic physics experiments?
The Department of Energy has amplified its backing of foundational science research in recent decades, which partly explains its interest in DUNE. But it also happens to have a vested interest in understanding neutrinos. If their scientists can understand how neutrinos work, they—and the rest of us—can gain a much clearer understanding of nuclear reactions, including fusion and fission and radioactive decay (important processes for the DOE as it shoulders rather high-stakes responsibilities of designing and storing nuclear weapons and overseeing nuclear power generation).
That potential understanding remains a big if. Neutrinos have defied definition for nearly 100 years.
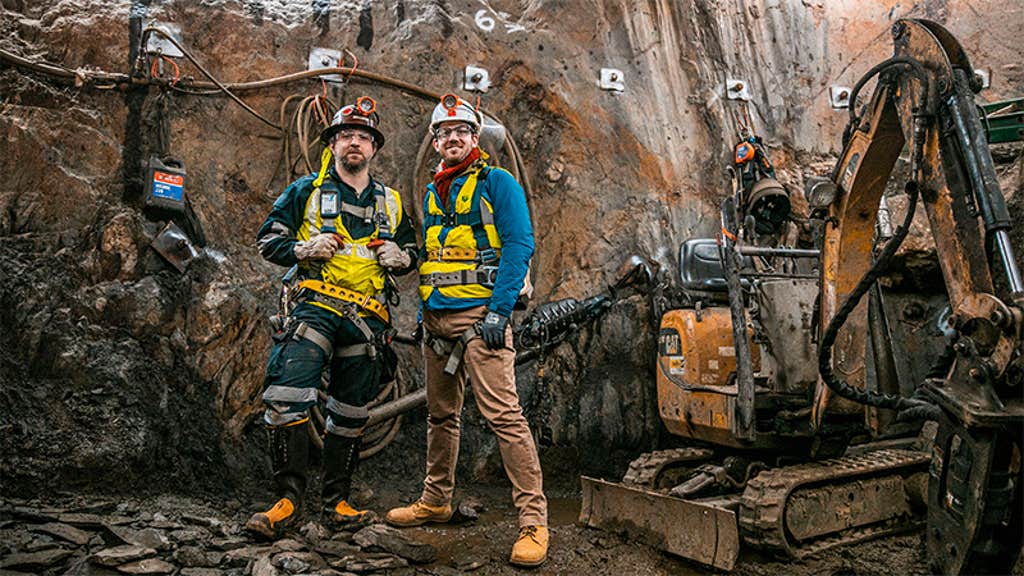
In the late 1920s, physicists studying a process called beta decay, in which a neutron spontaneously transforms itself into a proton and an electron, found those events didn’t entirely add up. Either their understanding of those reactions was askew, or some little trickster was invisibly carrying away a little bit of momentum. And thus they found traces of the neutrino: a massless, chargeless particle that only participated in a rare set of subatomic reactions.
Despite this discomfort—of not having predicted neutrinos’ existence with theory first—science plodded along, with the neutrinos mostly minding their own business, aside from some weird interfaces in various radioactive processes we could ascertain (such as the ferocious fusion reactions in the core of the sun). As experiments evolved, however, new problems emerged. Devices designed to measure the number of neutrinos pouring out of the sun only registered a fraction of what they were supposed to.
Once again, either we were misunderstanding something about nuclear reactions, or we were misunderstanding something about neutrinos.
To everybody’s chagrin, neutrinos were to blame. It turns out that they weren’t massless after all, nor were they uniform entities, nor were they stable, nor were their masses consistent. As they fly from the sun’s core, they can flip from electron-neutrinos to muon-neutrinos to tau-neutrinos—and even back again—their masses oscillating along the way.
A 2021 review revealed that DUNE was over budget and behind schedule.
We haven’t the faintest idea how or why these shapeshifters do what they do. But getting to the bottom of that could yield more precise research and applications in nuclear physics (DOE-speak for weapons and energy).
The problem lies in catching these dang particles to study. Frustratingly, neutrinos are everywhere. There are trillions—literally, trillions—of neutrinos coursing through your body every single second. We are awash in an endless sea of them. Despite those dizzying numbers, however, if you’re lucky, you might—might—interact with one in your entire life.
And that is because they barely even count as existing. The charge-free particles do not partake in the electromagnetic or strong nuclear forces. The current upper limits on their masses put them at something like 500,000 times lighter than an electron, and electrons are really tiny. This all makes actual interactions with them exceedingly rare. So rare that decades of searching and billions of dollars have managed to capture only a handful of them to learn from so far.
The Super-Kamiokande detector, which came online in 1996, had a startup cost around $100 million. Housed in Japan, Super-K (as it’s affectionately known) uses 50,000 metric tons of ultrapure water to try to catch neutrinos, to varying success. (In 1987 its predecessor observatory detected a burst of neutrinos from a distant supernova—and by “burst” I mean 12 particles.) An upgrade to Super-K, called appropriately enough Hyper-K, is now slated at half a billion dollars.
In Antarctica, the IceCube array uses kilometer-long strings of sensors drilled into the South Pole’s ice sheet. With a construction cost of $279 million, plus more to keep running it, in the 12 years since IceCube started science operations, it has captured a grand total of about 100 high-energy neutrinos.
We haven’t the faintest idea how or why these shapeshifters do what they do.
All of this is perhaps why funders, namely the Department of Energy, are taking a close look at DUNE’s slow, expensive trajectory. DUNE, which would beam neutrinos from Fermilab to Lead, South Dakota (home of the former Homestake experiment which originally studied solar neutrinos), promises the current holy grail of neutrino physics: understanding the masses of neutrinos, understanding deep nuclear processes, and possibly even understanding the earliest moments of the big bang (which produced a lot of neutrinos). Results that could potentially unlock new understandings of fundamental nuclear physics (and plenty of those other things the DOE is interested in). But a 2021 review revealed that DUNE was over budget and behind schedule.
Original plans had the operation scheduled for completion next year. Now that target is nearly a decade away. The original budget has ballooned to more than $3 billion. The DOE has deferred full approval of the project until sometime this year. After all, there are surely other promising avenues of science that could use a $3 billion boost. And perhaps scientists could find even cleverer, less heavyweight methods to probe the elusive neutrino.
As the future of DUNE hangs in the balance, pit half-dug, Argon detectors half-tested, it is just one of many current difficult conversations between physicists and the people that pay for their work. As we learn more about Nature, it becomes frustratingly difficult to learn even more. In response, science’s tendency so far has been to turn to ever-larger experiments to gather—and then sort through—massive quantities of data, hoping for a revelation. But funders are becoming more wary of big price tags. The James Webb Space Telescope famously cost nine times as much as originally planned, and finally arrived 15 years late. There’s no doubt the James Webb will reveal stunning new information about the cosmos, but only future generations might be able to assess if we got our $10 billion worth.
Basic physics experiments can be an even harder sell, especially when we haven’t made much progress on neutrino physics in decades. DUNE would likely reveal something new about these pesky little particles that have annoyed high-energy physics for almost a century. But the question is what—and would it be important enough to justify the accelerating cost?
Unfortunately the only way to find out would be to build it out and flip it on.
Alas, physicists are not in charge of the universe that we find ourselves in—or its budgets. In the midst of escalating costs and delayed schedules, with no hope of neutrino salvation in sight, we are forced to grapple with the big, uncomfortable question: What is scientific discovery worth?
Paul M. Sutter is a research professor in astrophysics at the Institute for Advanced Computational Science at Stony Brook University and a guest researcher at the Flatiron Institute in New York City. He is the author of Your Place in the Universe: Understanding our Big, Messy Existence.
Lead image: 35-ton-capacity Prototype cryostat for LBNF / DUNE, Anode Plane Assemblies – Construction of the DUNE 35-ton prototype detector. Credit: Reidar Hahn / Fermilab.