“The funny thing about life is that it’s temporary; that is to say, temporary in the ‘temporal’ sense of the word, meaning that all living things and all that we do are subject to the precepts and effects of time.”
—Lansing McLoskey, Theft
Many organisms perform best at certain hours of the day. The slug species Arion subfuscus, living in almost total darkness, knowing nothing about the Gregorian calendar, lays its eggs between the last week of August and the first week of September.1 Bees forage for nectar, knowing the best times to visit the best fields and the exact timing of nectar secretions for individual species of flowers.
In the mid-20th century, the Austrian Nobel laureate Karl von Frisch provided enormous insights on honeybee communication and foraging time. He discovered that bees have internal clocks that tell them not only where the nectar is to be found but also precisely when that food will be ready. “I know of no other living creature,” he wrote in his book on bee language, “that learns so easily as the bee when, according to its ‘internal clock,’ to come to the table.”2
Even without a light clue, the plants were able to tell time.
Indeed, honeybees start their daily routines of harvesting nectar by the clock or, rather, by sun time. While studying bee routines at his lab at the University of Munich before World War II, Frisch trained bees to come regularly to lunch at strategic times when feeding stations were set up with sugar water. The bees quickly adjusted their natural schedules to Frisch’s artificial schedule. In just two days their old schedule was abandoned. Even informational nectar-finding flights were stopped.
Judging from the conclusions of Frisch’s experiments, it seems as if physiotemporal processes in animals and insects rely on some internal rhythm that we could call an internal clock. Whatever it is, it must have some connection to the external events of sunlight and moonlight, the local time of earth’s turning and orbiting. Frisch’s student Martin Lindauer later corroborated and advanced Frisch’s experiments by hatching bees in a controlled environment of 12-hour daylight and 12-hour darkness. When the bees reached maturity they were trained to head in a given direction for at least five days.3 They learned the time of day purely from the sun’s position.4 Lindauer and Frisch were very much surprised, since at that time it was well known that birds use innate migration routes to navigate their journeys, and so do many insects. But a bee’s journey is more complex because it changes from day to day.
There is another tree with many leaves like the rose, and that closes at night, but opens at sunrise, and by noon is completely unfolded; and at evening again it closes by degrees and remains shut at night, and the natives say that it goes to sleep.”5 This quotation is a translation from the Greek that was written by Theophrastus of Eresos in the third century B.C. He was describing the daily leaf movements of the tamarind tree, suggesting that it is an organism whose physiology follows and responds to the time of day without any external cues, such as exposure to sunlight.6 Of course, we know now that all organisms have zeitgebers to help them adjust to seasonal variations of daylight, and so the tamarind surely had help from hidden cues. Buds appear without fail in the first week of April on a lilac bush on the south side of my house. The temperature could be close to freezing, and yet those buds will poke through as if to say, I sense the sun’s altitude angle is at about 51 degrees at noon, and that’s enough daylight for me to come out. Yay! Spring is coming! Now, where are those bees?
Experiments performed by the French astronomer Jean-Jacques de Mairan in 1729 established that plants have amazingly precise rhythmic behaviors that are independent of their environment. Mairan was interested in leaf movement and why certain plants, such as Mimosa pudica, a perennial weed found mostly in Central America, South America, and Asia, spread their leaves in daylight and fold them at night. Mairan recorded the mimosa’s leaf behavior under controlled conditions of constant darkness. Every day, in total darkness, the Mimosa pudica leaves opened during daylight hours and closed at almost the same exact time every evening. Much is now known about these orderly rhythms. First there is the sensitivity to temperature cycles. Mairan’s experiments with light did not account for temperature and moisture variations. The French physiologist Henri-Louis Duhamel du Monceau, who repeated Mairan’s experiments in 1758 with as much control as possible, placed the plants in blankets in a very temperate wine cellar. Despite all these isolations, the plant movements continued.7 Even without a light clue, the plants were able to tell time.4 In 1832 the Swiss botanist Augustin Pyramus de Candolle discovered that after the Mimosa pudica remained in darkness for a few days, its leaves would open about one or two hours earlier. The plant seemed to be adjusting its rhythm to its old routines with the sun, but its leaves’ sleeping period never went below a 22-hour cycle. Later in that century, Charles Darwin also got into the act while writing The Power of Movement in Plants, a book about leaf exposure to the sun and night. He wrote that natural selection favored these “sleeping plants’” ability to protect themselves from the cold of night with the benefit of sun absorption during the day.8
Even with the support of Monceau and Darwin, biologists did not fully embrace a belief in endogenous clocks. Some asked for a cause. Others asked for more convincing evidence that unforeseen environmental factors were not contributing to the phenomena. Then, in 1930, the German biologist Erwin Bünning experimented with Phaseolus, a native American wild bean plant, in total darkness and in total control of uniform temperature. He found that Phaseolus had a 24-hour cycle that did not coincide with any variations of the environment; therefore, the plant had an endogenous clock mechanism that controlled the folding and unfolding of its leaves. He experimented with red light to study its effects on leaf movement in marigolds and found an extremely accurate daily rhythm without any apparent cue from their environments. It seems that some plant rhythms have an inherited internal time scale that either obeys or competes with day-to-day light durations.
Insects, too, have internal daily rhythms. If your home has ever been invaded by fruit flies, you know how annoying those tiny buggers can be. For every one you whack or crush, 10 more will find you. What could be interesting about them? How much information about the world could come from those miniscule organisms the size of a poppy seed? It turns out that fruit flies share a significant percentage of genetics with humans, so they are model organisms for studying human-disease genes.
The complete mechanism of fruit fly genetics is as dauntingly difficult to explain as the depths of string theory. Fortunately, the outlying structure of at least the insect’s circadian clock can be fairly well understood without scaling the endless scaffolds of biochemistry, endocrinology, and physiology supporting the common genetics of fruit flies and humans. Early fruit fly molecular research at Cal Tech is documented in Ronald Konopka’s and Seymour Benzer’s landmark 1971 publication, which reports success in generating mutant genes that became arrhythmic.9 Leading chronologists claim that the work of Konopka and Benzer had an influence on the field of chronobiology that cannot be overstated, and their conclusions “were prescient for the entire circadian field and all of its subsequent molecular sophistication.”10
The simpler story, told here, is sure to be considered raw to the biochemists and entomologists that call the fruit fly a Drosophila melanogaster, though I expect it is the best that can be done within these few pages. So, without getting into the full description of the differences between protein functions of humans and flies, we can interpret the Drosophila model of the circadian clock as simply a feedback loop that operates by a specific gene expression with a relatively short half-life. In essence and in generality, the loop simply behaves like this: The quantity of A molecules increases, reaching a threshold that creates B molecules (with a relatively short half-life), which in turn shut down production of the A molecules.
Unlike fruit flies, humans have strong temperaments and wills that permit defiance of their weaker, yet persistent, biochemical controls. The model circadian rhythm for a person who rises at roughly 6 a.m. and runs through each day with a regularity tuned to the sun is broadly illustrated here.
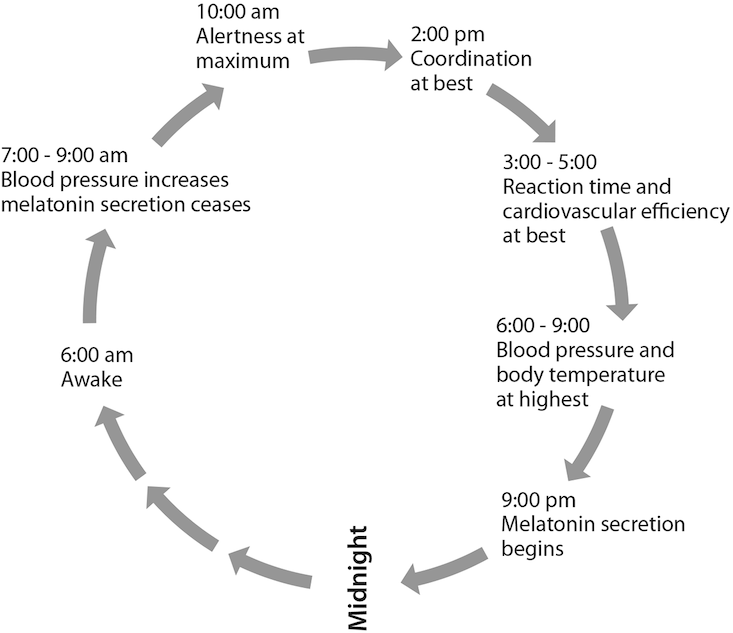
The human mind and body have a built-in circadian system, a coordinated assembly motivated to perform certain tasks, what we might call the macrobiological clock. At the molecular level there is a circadian oscillator, specific cell groups that work together, like the mechanism of a clock, to cause a larger system of mind and body to function in a daily rhythm. In the early 1980s Jeffrey Hall and Michael Rosbash, along with Rosbach’s graduate student Paul Hardin at Brandeis, discovered such a circadian oscillator in the fruit fly, an insect that has timekeeping gene qualities associated with clock genes in humans. Hall and Rosbash won the 2017 Nobel Prize in Physiology or Medicine for their discoveries of the molecular processes that control circadian rhythms. In their seminal paper in the Proceedings of the National Academy of Science, they isolated the so-called Period gene (per), which cycles the amount of messenger RNA (mRNA) produced in a feedback loop, first forming and then terminating proteins made from per gene instructions.11
For clarity, let’s first briefly recall the mechanisms of mRNA and proteins. The primary function of a gene, which is after all just a bit more than housing for a segment of DNA, is to give instructions for making protein molecules. Proteins, those chains of amino acids (chain groups containing oxygen, carbon, hydrogen, and nitrogen) responsible for maintaining and repairing cells, are made from genetic instructions transmitted from DNA to the ribosomes by messenger RNA. Ribosomes are intricate molecular machines that link amino acids together in the order specified by those instructions. The DNA in a cell’s nucleus stores all the gene instructions for copying specific segments of DNA into RNA that are essential for continued life. The mRNA within the nucleus leaves the nucleus and enters the cytoplasm to dictate information stored in the genes.
The complete mechanism of fruit fly genetics is as dauntingly difficult to explain as the depths of string theory.
All living things learn to manage daily environmental changes, especially the atmospheric lightness and darkness caused by the 24-hour cycle of earth rotation. A human’s hereditary information includes the biochemical mechanisms of proteins gotten from the routines of his or her ancestral life. And although millions of cells in a person’s body have specialized functions, every one of them contains the same code of hereditary information.
Ever since 1992, when Hardin, Hall, and Rosbash published their findings on circadian oscillations, it has been known that fruit flies can tell time by their per gene’s instructions. This led to the idea that a circadian gene’s instructions are responsible for per mRNA cycling following a feedback loop with return response instructions. The magic here is that the per gene located on the X chromosome of the fly cell contains the information for the mRNA (that has a relatively short half-life), which instructs the ribosomes to produce proteins connected with the per gene (called PER molecules, capitalized to avoid confusion with the per gene) that effectively travel back to the cell nucleus to turn off the activity of the per gene. Morning light would then destroy PER molecules. With PER molecules gone, the per gene would renew the process of encoding mRNA to complete a 24-hour feedback loop. In effect, it is the fruit fly’s molecular clock hand encapsulated in a single cell; moreover, it has since been discovered that the biological clock in most mammals works by the same feedback loop, though in mammals it takes a whole group of per genes for the process to continue. It could be that this Drosophila melanogaster per gene model is the result of organic evolutionary adaptation of the Earth’s circadian environment to maximize survival and well-being on a planet where life existence is governed by the alternation of light and darkness.
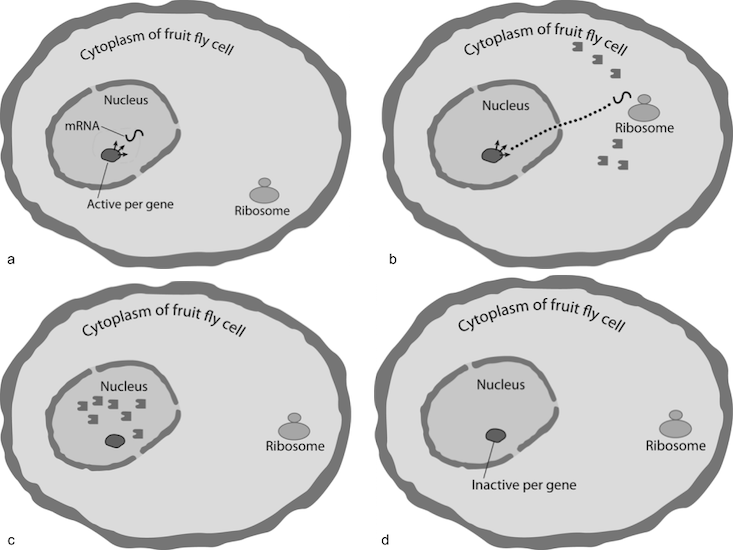
Here is how the circadian oscillator of Drosophila melanogaster works. The per gene in the nucleus of the cell transcribes mRNA molecules that migrate to the cytoplasm to give information and green light for ribosomes (the protein workshop) to build both stable and unstable protein molecules. The stable protein accumulates in the cytoplasm. As night goes on, protein levels accumulate to reach a threshold by roughly the middle of the night, when they enter the nucleus and begin to repress transcription from per gene instructions and soon after completely turn protein building off. In the morning, as the sun rises, the proteins decay and after several hours vanish. With all protein gone from the nucleus, the per gene turns on to restart transcription, and so the approximate 24-hour-cycle loop begins again. On and on it goes, indefinitely.
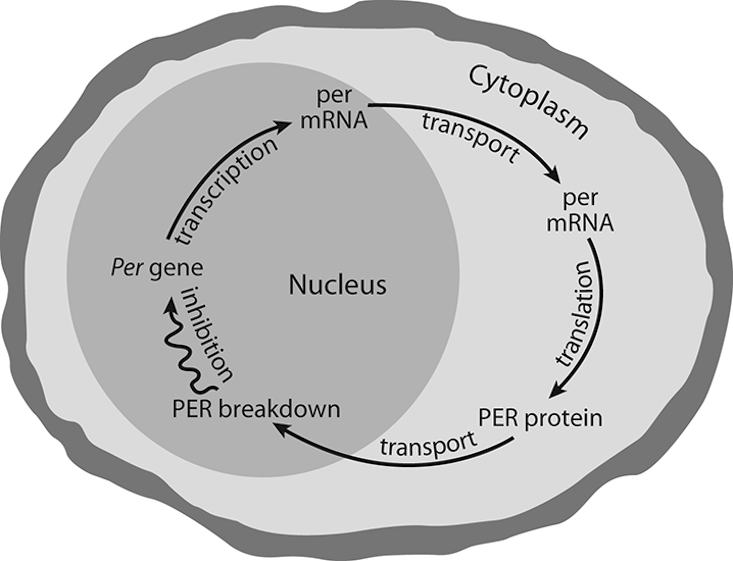
The frequency of oscillation is entirely controlled by the rate at which proteins accumulate in the cytoplasm, the rate at which the entire threshold group of proteins move to the nucleus, and the rate at which proteins break down once in the nucleus. Those rates match up to provide a full 24-hour loop, even without external cues. Perhaps that is why fruit flies hatch in highest numbers at dawn.12 And also perhaps, this is why human sleep patterns tend to conform to ordered sleep times and why any disruption of the order creates confusion in subsequent sleep periods.
Many living organisms, from snow fleas to Mimosa pudica, have evolved internal clock mechanisms that synchronize behavior, metabolism, and physiology with circadian rhythms. Humans, too, have specific cells that work as clock mechanisms tuned to circadian rhythms, but their oscillators are far more complex.13 Here is what is known. First, the Drosophila model is now acknowledged to parallel molecular mechanisms in humans, and since Drosophila and humans have functional homologues of most parts of human disease genes, the Drosophila model is enormously effective for research into human diseases and drug discovery. It tells how the cell is attached to the night-day cycle of sleep, melatonin and endocrine activity, cardiovascular changes, body temperatures, blood pressures, immune differences, and renal functions.
Humans are programmed to be diurnal, active in the daytime and inactive (or less active) at night. That’s a 24-hour behavioral and physiological rhythm that expects environmental conditions associated with the rotation of the earth. Of course there are larks and owls because we are, after all, humans, all a bit different and off the charts of rigid controls. In 1994 Joseph Takahashi and his lab teams at Northwestern University and the University of Wisconsin used the fruit fly model to search for time genes in mammals, finding and identifying them in mice.14 They identified the gene and named it CLOCK.1
Ensuing work established roughly homologous parallels between clock cell mechanisms in fruit flies and mammals. Mammals have three homologues to per, two of which produce clock proteins. Here is where things get too complicated for the scope of this article.15 However, there are some reasonably easy things to be said about mammals that should help us appreciate the bigger picture.
We are a bundle of clocks that are synchronized to the environment.
In 1972 the neurologists Robert Moore and Nicholas Lenn at the University of Chicago used amino acid tracers to identify a light-information route from the retina to the suprachiasmatic nucleus (SCN), suggesting that light cues entertaining circadian rhythms came from that pathway.16 In that same year Friedrich Stephan and Irving Zucker at Berkeley showed that lesions in the SCN of rats disturbed their circadian activity and drinking rhythms.17 Seven years later Shin-Ichi T. Inouye and Hiroshi Kawamura’s work with rats at the Mitsubishi-Kasei Institute of Life Sciences in Tokyo confirmed without doubt that, for rodents, electrical activity in the SCN followed circadian rhythms and that the SCN nucleus is an autonomous circadian pacemaker playing a major circadian role.18 Another confirmation happened in 1990, when Michael Menaker and his lab at the University of Virginia transplanted an SCN from a mutant golden hamster with a considerably shortened circadian rhythm of 20 hours and 12 minutes to a nonmutant hamster missing its SCN. When the receiving hamster’s circadian rhythm registered at 20 hours and 12 minutes, it became demonstratively clear that the SCN contains the pacemaker of mammalian circadian rhythms. The new rhythm could only be attributed to the transplanted SCN. In fact, restored rhythms seemed always to maintain the donor frequencies.19 The role of ocular photoreceptors and synchronized endogenous rhythms stemming from light signals transmitted to the SCN became indisputable.
We now know that all cells, including those deep in the center of the body, can maintain an autonomous frequency of oscillations. Each cell holds its own clock, and each of those clocks is driven by the same feedback loop that guides the pacemaker clock in the SCN. In mammals that pacemaker indirectly detects neural signals of light and darkness from the eyes to play a central role in regulating the body’s circadian activity rhythms through sleep-wake cycles.20 So the full body-clock system involves both the SCN in the brain and the trillions of peripheral clocks embedded in almost every cell of the body. We are a bundle of clocks that are synchronized to the environment by zeitgebers, of which changes in light and darkness are but just one.
With exception to cells in the eye, mammalian cells have no photoreceptors, so only the SCN can indirectly sense light by way of neural tract signals coming from the retina, and therefore, we tend to be awake mostly when light signals tell us to be. With normal and regular sleep-wake schedules the feeding cycle helps to align hormonal activities and to synchronize clocks in liver and intestine cells. Under noncircadian or restricted feeding schedules, the clocks of liver cells simply follow the feeding cycle, ignoring SCN calibration signals.
We might think that we have control over time’s grip on our will and behavior, that time has some feeble influence over body functions vulnerable to willful suppression, and that biological links from the mind and body to the external circadian cycle are too delicate to be taken seriously. Not true. The biochemical and genetic structures seem to be stronger than we suppose. They dictate time, by way of cell-to-cell transcription/translation feedback loops under endogenous control, in synchrony with extracellular geophysical cycles, to bring about changes in the behavior of the entire organism. Surprisingly, the loop continues through a feedback circle from behavior back to the molecular clock.21
The healthy human body has many feedback loop mechanisms that signal functional information from when to stop eating to when to rest. If we eat too much, the hormone leptin (an energy regulator) is produced to trigger a bloated feeling. Cells need to absorb nutrients, and they do so in a circadian rhythm of taking in nourishments by day and shutting down at night. In the course of a life, cells die and are replaced. That sequence happens with scars of the skin. Small rips of tissue are replaced with regrown cells. The same happens with cells well within the interior of the body and organs, where the breakdown and replacement cycle understandably coincides with the rhythm of sleep and wake times.
That breakdown and replacement cycle is just now being understood in the drug industry, where medications are being discovered to have optimum effects at particular periods of the 24-hour day and to have catastrophic effects at other periods. The consequences of rhythmic nature of individual cell functions could be a direct benefit for medication management and cancer radiotherapy.2 Particular timings could enhance or diminish or rescind the efficacy of a medication and in some cases could result in serious danger to the patient, possibly even death.22 Such seriousness has spawned a fresh pharmacological field, chronopharmacology. Like trains, our bodies run on a timetable.
Joseph Mazur is professor emeritus of mathematics at Marlboro College. His previous books include Euclid in the Rainforest: Discovering Universal Truth in Logic and Math and Fluke: The Math and Myth of Coincidence. He lives with his wife Jennifer in Vermont.
Excerpted from The Clock Mirage: Our Myth of Measured Time. Published by Yale University Press, with a release date in April. Reproduced with permission of Yale University Press. All rights reserved. No part of this excerpt may be reproduced or reprinted without permission in writing from the publisher.
References
1. Brett, J.A. The breeding seasons of slugs in gardens. Journal of Zoology 135, 559–568 (1960).
2. von Frisch, K. The Dance Language and Orientation of Bees translated by Chadwick, L.E.; Harvard University Press, Cambridge, MA (1967).
3. Lindauer, M. Time-compensated sun orientation in bees. Cold Spring Harber Symposium of Quantitative Biology 25, 371–377 (1960).
4. Whitrow, G.J. The Natural Philosophy of Time Clarendon Press, Oxford, United Kingdom (1980).
5. Theophrastus, Enquiry into Plants and Minor Works on Odours and Weather Signs translated by Sir Arthur Hort; C. P. Putnam’s Sons, New York, N.Y. (1916).
6. Schwartz, W.J. & Daan, S. “Origins: A Brief Account of the Ancestry of Circadian Biology,” in Kumar, V. (Ed.) Biological Timekeeping: Clocks, Rhythms and Behaviour Springer India, New Delhi (2017).
7. de Mairan, J. “Observation botanique,” in Histoire de l’Académie Royale des Sciences avec les Mémoires de Mathématique et de Physique Tirés des Registres de Cette Académie (1729).
8. Darwin, C. & Darwin, F. The Power of Movement in Plants D. Appleton, New York, N.Y. (1881).
9. Konopka, R.J. & Benzer, S. Clock mutants of Drosophila melanogaster. Proceedings of the National Academy of Science 68, 2112–2116 (1971).
10. Rosbash,M. “Ronald J. Konopka (1947–2015),” Cell 161, 187–188 (2015).
11. Hardin, P.E., Hall, J.C., & Rosbash, M. “Circadian oscillations in period gene mRNA levels are transcriptionally regulated. Proceedings of the National Academy of Sciences 89, 11711–11715 (1992).
12. Pittendrigh, C.S. The Harvey Lectures Academic Press, New York, N.Y. (1961).
13. Dunlap, J.C., et al. Light-induced resetting of mammalian circadian clock is associated with rapid induction of the mPer1 transcript. Cell 91, 1043–1053 (1997).
14. Vitaterna, M.H., et al. Mutagenesis and mapping of a mouse gene, clock, essential for circadian behavior. Science 264, 719–725 (1994).
15. For a deeper discussion, see Bechtel, W. & Abrahamsen, A., “Decomposing, Recomposing, and Situating Circadian Mechanisms: Three Tasks in Developing Mechanistic Explanations,” in Leitgeb, H. & Hieke, A. (Eds.) Reduction: Between the Mind and the Brain Ontos, Frankfurt (2009).
16. Moore, R.Y. Retinohypothalamic projection in mammals: A comparative study. Brain Research 49, 403–409 (1973).
17. Stephan, F.K. & Zucker, I. Circadian rhythms in drinking behavior and locomotor activity of rats are eliminated by hypothalamic lesions. Proceedings of the National Academy of Sciences 69, 1583–1586 (1972).
18. Inouye, S.-I.T. & Kawamura, H. Persistence of circadian rhythmicity in a mammalian hypothalamic ‘island’ containing the suprachiasmatic nucleus. Proceedings of the National Academy of Sciences 76, 5962–5966 (1979).
19. Ralph, M.R., et al. Transplanted suprachiasmatic nucleus determines circadian period. Science 247, 975–978 (1990).
20. Ko, C.H. & Takahashi, J.S. Molecular components of the mammalian circadian clock. Human Molecular Genetics 15, R271–R277 (2006).
21. Reebs, S.G. & Mrosovsky, N. Effects of wheel running on the circadian rhythms of Syrian hamsters; entrainment and phase response curve. Journal of Biological Rhythms 4, 39–48 (1989).
22. Halberg, F., et al. Transdisciplinary unifying implications of circadian findings in the 1950s. Journal of Circadian Rhythms 1, 1–61 (2003).
Lead image: VectorMine / Shutterstock